Research Article
Volume 2 Issue 1 - 2020
Feed Deprivation effects on Bactericidal an Immunological activity of Blood and skin mucus, and on blood Chemistry of Gilthead Seabream (Sparus aurata)
Dept. of Cell Biology and Histology, Faculty of Biology, Campus Regional de Excelencia Internacional “Campus Mare Nostrum”, University of Murcia, 30100, Murcia, Spain.
*Corresponding Author: María Ángeles Esteban, Dept. of Cell Biology and Histology, Faculty of Biology, Campus Regional de Excelencia Internacional “Campus Mare Nostrum”, University of Murcia, 30100, Murcia, Spain.
Received: May 31, 2020; Published: June 10, 2020
Abstract
Aquaculture needs to optimize production and nutrition of fish. The effects caused by 1, 2, 4 and 20 days of starvation on humoral immunity and bactericidal activity, against Vibrio anguillarum, in blood and skin mucus of gilthead seabream (Sparus aurata) were determined. In addition, several blood biochemical parameters were also analysed in order to test the general health status of the fish and also in an attempt to correlate the physiological changes due to starvation with the changes observed in the immune parameters. Significant decreases in IgM level and protease activity (P-value < 0.05), as well as significant increases in peroxidase and bactericidal activity were obtained in the mucus of the fish after short periods of starvation (from 1 to 4 days), compared to the fed fish. However, it was impossible to determine similar conditions in fish subjected to 20 days of starvation, since all the parameters studied in this group showed very similar values to those obtained for control fish (fed). This could be due to the necessity for fish to recover their homeostasis in order to survive, even when maintained under starvation conditions. The possible relationships between the nutritional state and humoral immunity in fish is discussed.
Keywords: Feed deprivation; Gilthead seabream (Sparus aurata); Immunity; Skin mucus; serum.
Abbreviations: 1D: 1 Day of feed deprivation; 20D: 20 Day of feed deprivation; 2D: 2 Day of feed deprivation; 4D: 4 Day of feed deprivation; ALB: Albumin; BA: Biliar Acids; BSA: Bovine serum albumin; CA: Calcium; DMSO: Dimethylsulfoxide; ELISA: Enzyme-linked immune sorbent assay; GLOB: Globulin; GLU: Glucose; HBSS: Hanks' Balanced Salt Solution; IgM: Immunoglobulin M; MINECO: Ministry of Economy and Competitiveness; MTT: 3-(4, 5 dimethyl-2-yl)-2, 5-diphenyl tetrazolium bromide; NA+: Sodium; OD: Optical density; PBS-T: Phosphate buffer and Tween; PHOS: Phosphate; SE: Standard Error; SGR: Specific growth rate; SHP: Small heterodimer partner; TCA: Trichloroacetic acid; TMB: 3,3’,5,5’-tetramethylbenzidine hydrochloride; TP: Total Protein; UA: Uric acid; Wspecific: Percentage of specific weight
Introduction
The current increase in world population is evident, and in order to maintain per capita consumption of seafood. With the objective of increasing production, maintaining the available space, it will be necessary to increase the efficiency of crops, and for that will be carried out various practices, formulate more efficient feed, increase the number of fish per tank, reduce mortality, and so on. Today, aquaculture production species dependent on feed amount to 51 million tonnes (Hasan, 2017). Aquafeed accounts for about 50-80% of aquaculture production cost and therefore its use has to be carefully considered and managed (Hasan, 2017).
Current aquaculture often involves intensive culture, resulting in severe overcrowding of individuals. Under these conditions, many specimens in a unit, such as a cage, strengthen their hierarchy, so that some groups could remain without feeding for short periods of time (Cerdá, 2012). Most farmed fishes are fed dry feed, industrially produced with different proportions of the main nutrients, depending on the dietary needs of the species. The main feeding system in marine cages is through pneumatic cannons in mobile boats. However, automatic feed distribution systems with video cameras and feed-fall detectors are increasingly being used to ensure that all fishes are being fed correctly (Love, 1980). If the feeding systems are defective, the fishes could starve over a period of time. It is known that many fish species can survive very long periods of feed deprivation but very quickly undergo changes in several biochemical and pathological parameters (Woo et al., 2011). Furthermore, diseases caused by nutritional deficiency appear when the concentration of a specific nutrient, obtained from the diet, falls below critical levels. Multiple nutritional deficiencies, common in many farmed fishes can generate undefined changes during long periods of feed deprivation. Infectious diseases or low nutrient uptake are often a consequence of a poor balanced diet (Mohapatra et al., 2015). Given the economic importance of fish feeding in aquaculture and knowing the significance of the immune system to preserve the wellbeing and health of the animals, it is essential to know how different periods of feed deprivation affect the activity of the main immune parameters. It has been demonstrated, however, that short periods of feed deprivation in aquaculture result in improved disease resistance and tolerance to handling and transport stress (Plisetskaya, 1980).
Several studies have examined in humans and fishes the relationship between feed deprivation and physiological and structural changes. Throughout the first days of feed deprivation, glycogen and later lipids are used as a source of energy (Sheridan and Mommsen, 1991). Gluconeogenesis then increases to generate enough circulating sugar in the blood. Once the liver’s energy reserves have been depleted, the fat stored in perivisceral lipid deposits begins to mobilize. The last tissue to be catabolized is the skeletal musculature, because during feed deprivation the protein turnover is very low due to the evolutionary pressure to conserve muscle mass in order to maintain tissue structure and function (Soeters, 2015).
Many studies have also been made to investigate general blood biochemistry, as well as the immune system of fishes, especially those of commercial interest (Folmar et al., 1992; Sandnes et al., 1988; Wedemeyer and Yasutake, 1977; Tietz, 1976; Lennox, 1924; Izar, 1911). However, to the best of our knowledge, there are no data about the changes in skin mucus immunity in starved fishes. The skin mucus of teleosts has several characteristics: it has intraspecific communication functions (Saglio and Blanc, 1989), reduces the force of friction in turbulent waters (Rosen and Cornford, 1971) and protects against parasites, fungi and bacteria (Pickering, 1974; Cameron and Endean. 1973). It is well documented that skin mucus contains many substances involved in immunity (Guardiola et al., 2015; Guardiola et al., 2016; Mercado et al., 2018; Khansari et al. 2018). More recently, it has also been used to determine fish stress (Guardiola et al. 2018). In addition, the study of skin mucus has certain advantages over serum as a biomarker of the state of fish, since its collection is minimally invasive, simple and rapid (Khansari et al., 2018; Palaksha et al., 2008). We therefore thought it was important to determine whether there was a direct correlation between the nutritional status of fishes and the immune parameters present on skin mucus. While severe stress can result in massive mortalities, sublethal stress can compromise various physiological and behavioural functions, leading to suppressed disease resistance and growth rate, both contributing to suboptimal production. The recognition of stressed states, as well as the management of fish health are therefore critical to the success of an aquaculture operation (Hasan, 2017).
Taking into account all these considerations, the aim of the present research was to test if there were any significant variations in the biochemical and immune parameters in serum and skin mucus of gilthead seabream (Sparus aurata L.) related to different periods of feed deprivation, this would then ascertain whether the mucus could be used as a good indicator of nutritional status. Gilthead seabream were used because is one of the most farmed marine fish species at present and non-lethal and few-invasive techniques were selected according to the 3Rs (replacement, reduction and refinement) principles.
Materials and Methods or Experimental Procedures
Fish:One hundred juvenile gilthead seabream of 125 ± 25 g body weight (mean ± S.E.) were obtained from a local farm (Murcia, Spain) and randomly distributed in 10 tanks (10 fish/thank) of sea water (250 L) at the Marine Facilities of the University of Murcia. The water was maintained at 20 ± 2ºC, 28 ‰ of salinity and circulated at 900 L/h. The fish acclimatized for 2 weeks under these conditions and were fed daily at a rate of 2% of their mean tank biomass with a commercial diet (Skretting, Spain; www.skretting.com/es-ES/). After the acclimatization period, fish in two tanks continued being fed in the same way (control group), while those of the other tanks were subjected to different time regimes of feed deprivation, in duplicate. Before starting the experiment and at the end of each feed deprivation period (days), the specimens were weighed (g) to calculate the specific growth rate (SGR); SGR = 100 (ln WFinal – ln WInitial)/(tFinal–tInitial), where WInitial = initial weight, WFinal = final weight, tInitial = initial time and tFinal = final time, and the percentage of specific weight (WSpecific): WSpecific = 100(WFinal– WInitial)/(WInitial (tFinal–tInitial)). All the experimental protocols were approved by the Commission of Ethics of the University of Murcia.
Experimental design and sampling: All the fish in two tanks were sampled after each period of feed deprivation (n=20); 1 (1D), 2 (2D), 4 (4D) and 20 days (20D), and control (Control). We selected this fasting period because fish could remain for certain short periods of time without feeding and because the main biochemical changes are evident in these periods (Hasan, 2017). Before sampling, the fish were anaesthetized with clove oil (Sigma; 5:100 v/v marine water) and aliquots of 1 mL of skin mucus was collected with a rubber spatula by using gentle dorsal-lateral massage on both sides of the body (Palaksha et al. 2008), for each fish. The samples were then centrifuged (250 x g, 10 min, 4ºC) and the concentration of total protein present in the supernatants of each sample was determined by the Bradford method (Bradford, 1976), using bovine serum albumin (BSA, Sigma; www.sigmaaldrich.com) as a control. The protein concentration of the mucus samples was adjusted to 500 mg of protein/mL with Milli-Q water. The samples were then stored at -80 ºC until analysed.
Aliquots of 1 mL of blood were also obtained from the caudal vein of each specimen. The blood samples were cooled to 4ºC for 4 h before being centrifuged (11110 x g, 10 min, 4ºC). Then the serum was collected and the protein concentration was determined in the same way as described above. The serum samples were also stored at -80ºC until analysed.
Biochemical parameters:Three serum samples per treatment were analysed with the VetScan VS2 Chemistry Analyzer (Abaxis; www.abaxis.com), using the Avian/Reptilian Profile Plus (Abaxis) commercial rotors. Concentrations of bile acids, glucose, uric acid, total protein, globulin, albumin, calcium, phosphate and sodium were measured.
Immune parameters
Immunoglobulin M (IgM):To measure IgM levels, an enzyme-linked immune sorbent assay (ELISA) was performed following the protocol proposed by Guardiola et al. (Guardiola et al., 2014). Dilutions were made to add 10 µg, in triplicate, of mucus or serum proteins on 96-well flat bottom plates and incubated (one night, 4 ºC) with 100 µL of carbonate-bicarbonate buffer (50 mM, pH 9.6). After three washes with 100 µL of PBS-T (phosphate buffer 0.1 M and 5:10000 v/v Tween 20, pH 7.3) per well, the plates were blocked for 2 h at room temperature with a blocking buffer containing 0.03 mL/L of BSA in PBS-T, followed by three new washes with PBS-T. The plates were then incubated for 1 h with 100 µL per well of anti-gold monoclonal IgM mouse antibody (Aquatic Diagnostics Ltd; http://aquaticdiagnostics.com/) (1:100 in blocking buffer solution), washed and incubated with the secondary antibody (Anti IgG mouse made in goat, dilution 1:1000 in blocking buffer solution, Sigma). After thorough washing with PBS-T, the process was continued using 100 µL of a 0.42 mM solution of 3,3’,5,5’-tetramethylbenzidine hydrochloride (TMB, Sigma), prepared at the time in a 100 mM citric acid/sodium acetate buffer (pH 5.4) containing 1:10000 v/v of H2O2. The reaction was left to act for 10 min and stopped by adding 50 µL of 2 M H2SO4. The plates were read at 450 nm with a plate reader (FLUOstar Omega, BMG Labtech; https://www.bmglabtech.com/fluostar-omega/). Negative controls consisted of samples without cutaneous mucus or serum or without primary antibodies, whose optical density (OD) values were subtracted.
Immunoglobulin M (IgM):To measure IgM levels, an enzyme-linked immune sorbent assay (ELISA) was performed following the protocol proposed by Guardiola et al. (Guardiola et al., 2014). Dilutions were made to add 10 µg, in triplicate, of mucus or serum proteins on 96-well flat bottom plates and incubated (one night, 4 ºC) with 100 µL of carbonate-bicarbonate buffer (50 mM, pH 9.6). After three washes with 100 µL of PBS-T (phosphate buffer 0.1 M and 5:10000 v/v Tween 20, pH 7.3) per well, the plates were blocked for 2 h at room temperature with a blocking buffer containing 0.03 mL/L of BSA in PBS-T, followed by three new washes with PBS-T. The plates were then incubated for 1 h with 100 µL per well of anti-gold monoclonal IgM mouse antibody (Aquatic Diagnostics Ltd; http://aquaticdiagnostics.com/) (1:100 in blocking buffer solution), washed and incubated with the secondary antibody (Anti IgG mouse made in goat, dilution 1:1000 in blocking buffer solution, Sigma). After thorough washing with PBS-T, the process was continued using 100 µL of a 0.42 mM solution of 3,3’,5,5’-tetramethylbenzidine hydrochloride (TMB, Sigma), prepared at the time in a 100 mM citric acid/sodium acetate buffer (pH 5.4) containing 1:10000 v/v of H2O2. The reaction was left to act for 10 min and stopped by adding 50 µL of 2 M H2SO4. The plates were read at 450 nm with a plate reader (FLUOstar Omega, BMG Labtech; https://www.bmglabtech.com/fluostar-omega/). Negative controls consisted of samples without cutaneous mucus or serum or without primary antibodies, whose optical density (OD) values were subtracted.
Protease:Protease activity was quantified by means of a hydrolysis test of azocasein (Hanif et al., 2004) according to Guardiola et al. (Guardiola et al., 2014). Samples of 10 µL of serum were incubated with 125 µL of azocasein (Sigma) at 2:100 w/v in NaHCO3 and 100 µL of NaHCO3 during 24h at room temperature and continuous agitation. Afterwards, 250 µL of TCA (trichloroacetic acid) were added and the samples were centrifuged (21770 x g, 5 min). Aliquots of 100 µL of each sample were transferred, to 96-well flat bottom plates in triplicate. Then, 100 µL of NaHCO3 per well were added and the OD was determined in a plate reader at 450 nm. The procedure to measure the protease activity in mucus started from a volume of 100 µL of sample and 125 µL of azocasein at 7:1000 w/v in NaHCO3. It then followed the same methods as for serum. In control samples, the volume of mucus or serum was substituted by trypsin (5 mg/mL, Sigma) for positive control (100% protease activity) or NaHCO3 as negative control (0% protease activity).
Antiprotease:Antiprotease activity was determined as the ability to inhibit the activity of trypsin by means of the azocasein hydrolysis assay (Hanif et al., 2004), according to Guardiola et al. (Guardiola et al., 2014). Aliquots of 10 µL of serum and 10 µL of trypsin were incubated for 10 min with agitation at room temperature. Then, 125 µL of 2:100 w/v azocasein were added in 100 µL of NaHCO3 and incubated for 2 h followed by 250 µL of TCA before centrifuging (21770 x g, 5 min). Then 100 µL of each sample was transferred, in triplicate, to 96-well flat bottom plates and 100 µL of NaHCO3 per well were added. Finally, the OD was determined in a plate reader at 450 nm. The procedure to measure the antiprotease activity of mucus was based on a volume of 100 µL of sample and 125 µL of 7:1000 w/v azocasein in NaHCO3 and then the method was the same as for the serum. For controls, the volume of mucus or serum was replaced by trypsin (5 mg/mL) for a positive control (100% protease activity) or NaHCO3 as a negative control (0% protease activity).
Peroxidase:Peroxidase activity in mucus (Quade and Roth, 1997) was determined according to Guardiola et al. (Guardiola et al., 2014). Previously, 30 µl of sample (mucus and serum) were diluted with 120 µl of Hank’s buffer (HBSS) in 96-well flat-bottomed plates. Then, 50 µl of TMB 20 mM and H2O2 5 mM were added and after 2 min, the reaction was stopped with 50 µl of H2SO4 2M. The OD was measured on a plate reader at 450 nm. The blank samples corresponded to samples without mucus or serum. One unit was defined as the amount that produced an absorbance change of 1 and the activity expressed as U/mg mucus protein or serum.
Bactericidal activity:The bactericidal activity in mucus was determined by evaluating its effect on bacterial growth curves (Sunyer and Tort, 1995). Vibrio anguillarum, an opportunistic marine pathogen (kindly donated by Dr. MA Moriñigo, University of Málaga, Spain), was used for evaluating bactericidal activity. The bacteria cells were grown at 25ºC on agar plates (0.015 g/mL) with soy triptone medium (TSB, Difco Laboratories; https://www.fishersci.com/us/en/brands/I9C8LUGQ/difco-laboratories.html) at a concentration of 30:1 w/v and 15:1000 w/v NaCl. Subsequently, isolated colonies were diluted in 5 mL of liquid culture medium and introduced into an orbital incubator (16 h, 25ºC, and 1 x g). Then, 20 µL of mucus or serum and 20 µL of bacterial dilution at a concentration of 106 bacteria/mL were added to each well of 96-well plates. The samples were cultured (5h, 25ºC). After incubation, 25 µL/well of MTT [3-(4, 5 dimethyl-2-yl)-2, 5-diphenyl tetrazolium bromide, 1 mg/mL, Sigma] were added and the samples were incubated for another 10 min (25ºC), before being centrifuged (2000 x g, 10 min). The supernatant was removed and the precipitate dissolved with 200 µL/well of dimethylsulfoxide (DMSO, Panreac). Aliquots of 100 µL from each well were transferred to a new plate of 96 flat-bottomed wells. Absorbance was then measured at 570 and 690 nm. PBS was used as a positive control (0% of bactericidal activity).
Statistical study: All the results are expressed as mean ± S.E. The data were analysed with the software IBM SPSS Statistic for Windows [IBM Corp (2014) Version 23.0.; https://www.ibm.com/SPSS-Statistics/] and the graphical representations were made with RStudio (https://www.rstudio.com/). The normality of the variables and the homogeneity of the variances were confirmed by the Shaphiro-Wilk and the Levene tests, respectively. An ANOVA of a single factor was used to determine statistically significant differences between the five treatments, assuming significance level of 95% (P-value < 0.05), followed by the Tuckey or Games Howell tests, according to the homogeneity of the variables.
Results and Discussion
In order to maintain the present consumption of seafood per capita for an increasing human population, it will be necessary to increase aquaculture production to 23 million tonnes by 2020 (Iwama, 1997). There are many variables that can cause stress to fishes including poor water quality (Schreck, 2000), non-optimal salinity or temperature (Carmichael et al., 1984; Carmichael et al. 1998), high fish density (Vijayan and Leatherland, 1998), inappropriate background colour (Gilham and Baker, 1985) and poor nutrition (Barton et al., 1998; Vijayan and Moon, 1992). The basic needs of most animals are similar and for this reason, many types of stressors are considered universal throughout the animal kingdom. Universal stressors include deviations from optimal ranges for environmental variables, inadequate refuge from sunlight or predators, negative social interactions such as territorial disputes and insufficient food availability. Farmed fishes reared in cages or tanks often experience several of these stressors in the form of handling, crowding, nitrogenous waste accumulation and suboptimal nutrition (Love, 1980; Mohapatra et al., 2015; Plisetskaya, 1980; Folmar et al., 1992). In the present study we focused on the consequences of suboptimal nutrition. To evaluate stress responses in ?shes, several methods can be applied (Pickering and Pottinger, 1989; Kane et al., 2004; Wendelaar, 1997; Barton, 2002; Ryan and Hightower, 1994). We selected whole-body weight (Barton, 2002; Ryan and Hightower, 1994), the biochemical composition of blood (Dutta et al. 2005; Hosoya et al., 2007; Acerate et al., 2004; Barton, 2002; Iwama et al., 2004; Olsen et al., 2008) and immune parameters (Trenzado et al., 2008) as few-invasive methods.
Before starting the experiment and at the end of each feed deprivation period, all fish in each tank were weighed, to calculate the average weight of the fish per tank and to determine possible variations within each tank. Morphometric relationships can be used to determine the nutritional status of a fish, but they vary with intrinsic parameters such as age and stage of development. In the present study no statistically significant variation in weights of the fish in groups 1D, 2D and 4D were observed compared to the weight of control fish. Significant differences from the fed, control fish were only detected in the 20D group, where statistically significant weight losses were recorded (Table 1).
Control | 1D | 2D | 4D | 20D | |
Wspecific (%) | 7.99 ± 4.38a | -0.02 ± 0.95ab | -0.67±1.23ab | -1.87 ± 1.45ab | -8.48 ± 0.99b |
SGR (%) | 0.34 ± 0.22a | -0.06 ± 0.95a | -0.36 ± 0.58a | - 0.49 ± 0.37a | -0.44 ± 0.05b |
Table 1: Mean ± S.E. percentage specific change in weight per day (Wspecific) and specific growth rate (SGR). for each feed deprivation period: control, 1 day (1D), 2 days (2D), 4 days (4D) and 20 days (20D) . The letters determine the statistical groups with significant differences between the different treatments (P-value < 0.05).
These short times have been selected for the present work because the farmed fish could be easily suffering feed deprivation for these short periods in farm conditions. During feed deprivation, the whole body or some organ sizes and weights can change (e.g. the condition factor and the hepato-somatic and gonado-somatic indices) (Ryan and Hightower, 1994; Dutta et al., 2005). Furthermore, muscle catabolism, many biochemical changes, and the mobilization of glycogen, lipids and proteins can take place (Mohapatra et al., 2015). However, these do not occur until very advanced stages of feed deprivation when muscle mass begins to be metabolised (Folmar et al., 1992). On the other hand, 20 days was selected as a period of feed deprivation that can be considered long. Thus in the present study no statistically significant loss of weight was observed until 20 days of feed deprivation. These results agree with those obtained in rainbow trout (Oncorhynchus mykiss L.) in which, similarly, weight loss began to be observed after 21 days of feed deprivation (Vijayan and Moon, 1992).
Glucose is the main substrate for cell and matrix maintenance, controlling the redox state. However, as a limitation of the irreversible oxidation of glucose the body begins to use fat as fuel, increases the oxidation of ketone and fatty acids and decreases the oxidation of glucose (Folmar et al., 1992). Lipid mobilization has also been considered a common response to feed deprivation in fishes (Choi et al., 2007; Kaneko et al., 2016). During short periods of feed deprivation, lipids provide energy for metabolic activities and maintain the homeostasis of the fish (Liao et al., 2017). It has also been shown that, in feed deprivation, the use of proteins is delayed as much as possible to maintain the structure of the organism and allow it to perform the most important metabolic functions (Folmar et al., 1992). The amino acids used during feed deprivation come from peripheral tissues to maintain storage in central organs. Thus, amino acids go from peripheral tissues to important central organs in order to not impede the generation of acute phase proteins and immune cells, cell proliferation and matrix regeneration (Folmar et al., 1992). As mentioned above, feed deprivation involves the mobilization of molecules and their catabolism in certain tissues. Thus, when cell membranes are intact, the enzymes are present in serum at low concentrations, since cell membranes are impermeable to them when the cell metabolizes normally (Wedemeyer and Yasutake, 1977). Several changes in the molecular composition of the serum, and possibly skin mucus, may occur with time of feed deprivation (Heming and Paleczny, 1987). Nine blood parameters, selected from those used generally in veterinary diagnosis, were measured in order to detect if there were any significant physiological variations with different feed deprivation times (Table 2). Although only statistically significant differences were found between the different experiment times for total proteins, a correlation was observed between changes in concentrations of all parameters studied with respect to the feed deprivation times.
During fasting, not all bile acids (BA) enter the gallbladder (Setchell et al., 2012) and while serum BA concentration is low in normal circumstances, BA increase after long periods of feed deprivation (Barnes et al., 1975). In the present study, the BA were not altered in 1D or 2D fish compared to the values of control group, but increased in those of the 4D group (Table 2). Bile acids inhibit the expression of gluconeogenesis genes, including glucose-6-phosphatase, phosphoenolpyruvate carboxykinase and fructose 1,6-bis-phosphatase in a form dependent on the nuclear receptor SHP (small heterodimer partner). This increase in BA in serum from fish of group 4D coincided with a slight decrease in serum glucose (GLU) in the same group, with respect to fish of group 2D, as a response to the phenomenon explained above. In the fish of groups 1D and 2D, compared to the control groups, there was an increase in the concentration of GLU possibly due to an early use of GLU as an energy source followed by hepatic gluconeogenesis (Woo et al., 2011). In fish from the 20D group, however, there was a decrease of GLU compared to the control group, perhaps due to the exhaustion of the reserves. A similar observation in Salmo trutta, in which after 28 days of feed deprivation there was a decrease of GLU in the serum (Heming and Paleczny, 1987).
Control | 1D | 2D | 4D | 20D | |
BA (µmoll/L) | 35.33 ± 0.67ab | 34.33 ± 0.33a | 35 ± 0.58ab | 49 ± 6.81b | 36 ± 0.57ab |
GLU (mgd/L) | 88.67 ± 6,98 | 135 ± 32.08 | 142.33 ± 24.26 | 126.33 ± 11.1 | 76 ± 12 |
UA(mg/L) | 0.2 ± 0 | 0.3 ± 0.1 | 0.27 ± 0.07 | 0.2 ± 0 | 0.27 ± 0.07 |
TP(gd/L) | 4.13 ± 0.09ab | 4.93 ± 0.39b | 3.8 ± 0.21a | 4.13 ± 0.12ab | 4.4 ± 0.23ab |
GLOB(gd/L) | 2.13 ± 0.03 | 2.23 ± 0.22 | 1.77 ± 0.12 | 2.1 ± 0.21 | 2.27 ± 0.18 |
ALB(gd/L) | 1.97 ± 0.07 | 2.87 ± 0.15 | 2.07 ± 0.15 | 2.03 ± 0.19 | 2.17 ± 0.09 |
CA(mgd/L) | 13.57 ± 0.19 | 15.37 ± 1.63 | 14.67 ± 1.17 | 13.67 ± 0.41 | 14.9 ± 0.4 |
PHOS(mgd/L) | 11.93 ± 0.27 | 15.47 ± 1.43 | 12.13 ± 1.05 | 10.6 ± 0.61 | 11.53 ± 1.05 |
NA+ (mmol/L) | 171 ± 0 | 181.67 ± 5.33 | 174.33 ± 3.33 | 173.33 ± 3.93 | 177.67 ± 3.33 |
Table 2: Mean ± S.E. values of bile acids (BA), glucose (GLU), uric acid (UA), total protein (TP), globulin (GLOB), albumin (ALB), calcium (CA), phosphorus (PHOS) and sodium (NA+) for each treatment : control, 1 day (1D), 2 days (2D), 4 days (4D) and 20 days (20D). Lower case letters denote the statistical groups with significant differences between the different starvation periods (P-value < 0.05).
Various studies have shown that feed deprivation increases the concentration of uric acid (UA) in the serum (Izar, 1911). Possible causes of this are catabolism of purines, hydraulic regulation by catabolizing proteins, accumulation of UA in blood (Izar, 1911) or reduction of urolitic activity during feed deprivation, as occurs in dogs (Saglio and Blanc, 1989), Uric acid concentration is considered to be a good indicator of renal status. The concentration of UA, compared to the control group, tended to increase only in serum from fish of the 1D group, as was also noted in GLU, in response to the degradation of purines. In contrast, a decreasing trend was observed in the serum from fish of groups 2D and 4D, while in the fish of group 20D, there was an increase in the concentration of UA, compared to control fish. This may be explained by in response to the decrease in GLU, perhaps due to liver malfunction, renal gluconeogenesis begins to occur from glutamine generated during renal catabolization. This mobilized glutamine passes to gluconeogenesis and purine formation for energy maintenance, which when degraded generates UA (Moyes and Schulte, 2007; Kardong, 2007; Cox and Nelson, 2014).
Serum proteins have many functions, including maintaining water balance in fishes (Tietz, 1976). Thus, total proteins (TP) are a parameter used in clinical veterinary medicine to determine nutritional and hydration status, as well as liver, kidney, muscle and digestive diseases. Changes in serum TP may result from the combination of changes in different protein fractions, and consequently can be tracked by an estimation of globulin (GLOB) and albumin (ALB). Globulin is a group of proteins used in diagnosis to determine antigenic stimulation and dehydration (Cox and Nelson, 2014). Serum proteins have different origins, but ALB is only synthesized in the liver (Wedemeyer and Yasutake, 1977). The ALB is a protein involved in processes of regulation of hydration and transport of substances through the bloodstream. Its presence in serum in high concentrations serves as a diagnosis of liver problems. In this way, in the present study, although only the TP showed statistically significant differences, the TP, GLOB and ALB showed similar trends of change varying with feed deprivation time (Table 2). The concentrations of TP, GLOB and ALB increased in serum from fish belonging to group 1D, compared to the control fish, perhaps as a consequence of the mobilization of the recently digested macromolecules. Similarly, decreases in proteins were observed in serum from fish of the 2D group, perhaps because the precursor macromolecules were no longer available, but the concentrations were too high to promote the use of reserves. However, some increases were observed in serum from fish belonging to the 4D group, perhaps as a result of the catabolization of reserves (Sheridan and Mommsen, 1991; Folmar et al., 1992), a trend that also was detected in serum from fish of the 20D group, compared to values recorded for control fish.
Among other functions, ALB is responsible for fixing serum calcium (CA). High concentrations of CA and phosphorus (PHOS) in serum may be due to a deficiency of vitamin D, which fixes CA and PHOS, or to renal insufficiency, since the ions are responsible for reabsorbing CA and eliminating PHOS. Likewise, other serum ions such as sodium (NA+) are used to determine renal status, malnutrition, tissue degradation and dehydration status because they are ions involved in the osmoregulation and active transport of cellular transmembrane substances (Moyes and Schulte, 2007; Kardong, 2007; Cox and Nelson, 2014).
Our results demonstrate that CA, PHOS and NA+ had a similar behaviour in the specimens of each treatment, so that, compared to the control group, the concentrations increased in the 1D group, tended to decrease in fish of the 2D and 4D groups and increased in fish of the 20D group.
The physiological changes that occurred in each treatment due to the periods of feed deprivation, to which the fish were subjected, were linked to possible correlations with seric activity changes involved in the immune system. Physiological changes noted in the serum were compared with the same activities determined in skin mucus, to validate their possible use as indicators of the nutritional state of the animals. Immunoglobulin M (IgM) is the first immunoglobulin to act when an immune response is generated (Murphy et al., 2008; Ellis, 1999). The IgM (a serum globulin) (Figure 1a) and the GLOB (Table 2) values obtained behaved in the same way, but only significant differences from the control group were observed in the fish from groups 1D and 2D. Furthermore, IgM levels in mucus (Figure 1a) behaved the same way as in the serum, although its concentration in mucus was always lower than in serum, which coincides with previous studies (Hanif et al., 2004). In addition, IgM levels in serum recovered to their normal values earlier (2D) than in mucus (4D). These results seem to suggest that systemic IgM levels recovered more rapidly than IgM in the skin although more studies are needed to understand IgM level changes.
Protease activity consists of the enzymatic ability to hydrolyze other proteins. The increments observed in serum protease activity (Figure 1b) in the different treatments, compared to control fish, indicated that protease was sensitive to changes in the normal status of the fish. In the present study, the highest value of seric protease activity was observed in fish serum of group 1D. Protease activity decreased in mucus from fish of groups 1D, 2D and 4D, indicating that the determination of protease activity in skin mucus could be a good indicator of nutritional status. On the other hand, there was a higher protease activity in serum than in skin mucus (Figure 1b). However, in a previous study, higher protease activity was detected in mucus than in serum (Hanif et al., 2004). These differences in results may be due to variability associated with the ability of poikilotherms to adapt to natural stress, such as the availability of food, as well as seasonal variations in the biochemical composition of the blood (Wedemeyer and Yasutake, 1977).
Fish plasma contains a lot of antiprotease activity due to the presence of α2-macroglobulin and α1-antiprotease (Ellis, 1999). Antiprotease activity was not detected in skin mucus in the present work, perhaps because we used a lower protein concentration of this matrix, compare to the previous study (Hanif et al., 2004). Antiprotease activity in serum in fish of groups 1D, 2D and 20D was lower than in serum from the control fish (Figure 1c). For this reason, and because of the low antiprotease activity in skin mucus, antiprotease cannot be considered as a good indicator of nutritional status.
Stress increases oxygen free radical (ROS) levels, negatively affecting lipids, proteins and DNA (Khansari et al., 2018). In addition, cell lysis during prolonged feed deprivation results in increased ROS in the bloodstream. In the present study, increased serum peroxidase activity was recorded in serum from fish of 4D group (Figure 2a), coinciding with the onset of the decline in serum GLU (Table 2). The increases detected in the peroxidase activity could be interpreted as a response to the change in serum redox status. On the other hand, peroxidase activity in skin mucus was also increased but in fish in groups 2D and 4D. These results support the idea that peroxidase activity in mucus could be considered a good indicator of nutritional status, mainly due to the fact that this activity seems to change earlier in mucus than in serum.
Finally, the bactericidal activity in serum and mucus V. anguillarum, a pathogenic marine bacterium that infects many aquaculture species of commercial interest (Harrel et al., 1976), was determined. Bactericidal activity only decreased in fish serum from the 1D group and increased in skin mucus of the 2D fish. The bactericidal activity against this pathogen could be considered as an indicator of the nutritional state in mucus, after short periods of feed deprivation. Present results demonstrated that both mucus and serum have high bactericidal activity against V. anguillarum (Figure 2b), which agrees with previous results (Hanif et al., 2004; Harrel et al., 1976). It is known that in skin mucus and also in serum there is a large concentration of antimicrobial peptides, that is very important for the survival of the fish (Guardiola et al., 2015). Previous studies (Fletcher and Grant, 1969) and our results determined that the mucus contained fewer macromolecular components than serum, and we deduced that the mucus must be involved in a specific secretion system of survival against infectious organisms.
In general, for all the enzyme activities measured both in serum and skin mucus, the values obtained in fish in group 20D were similar to those obtained in fish in the control group, except for protease and antiprotease activities in serum (Figuress. 1 and 2). A possible explanation for this could be an effort to maintain homeostasis in order to survive. It is known that organisms under stress can adapt to a new level of homeostasis in an attempt to increase cell survival. This same phenomenon was observed in BA, CA and PHOS seric levels in the present study (Table 2).
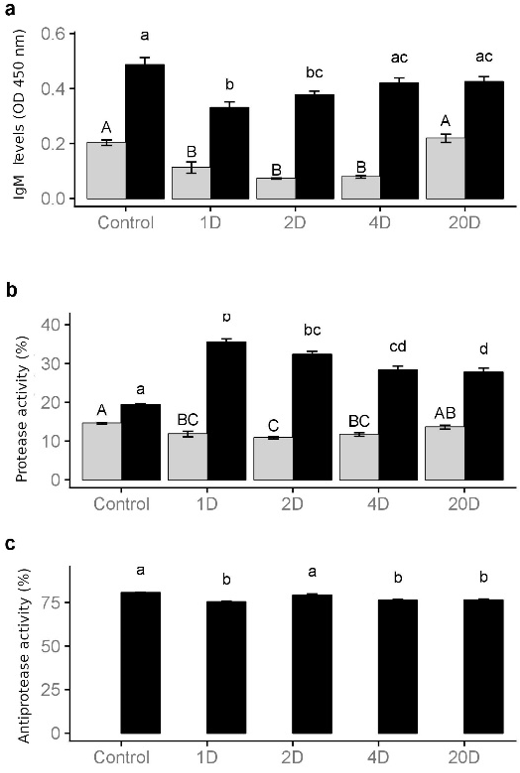
Figure 1: a) Immunoglobulin M (DO 450 nm), b) protease (%) and c) antiprotease (%) activity determined in serum (grey boxes) and mucus (filled boxes) samples of Sparus aurata subjected to different times of feed deprivation: control (fed), 1 day (1D), 2 days (2D), 4 days (4D) and 20 days (20D). Values are means ± S.E. (n=10). The letters above the bars denote the statistical groups with significant differences between the different treatments (P-value < 0.05).
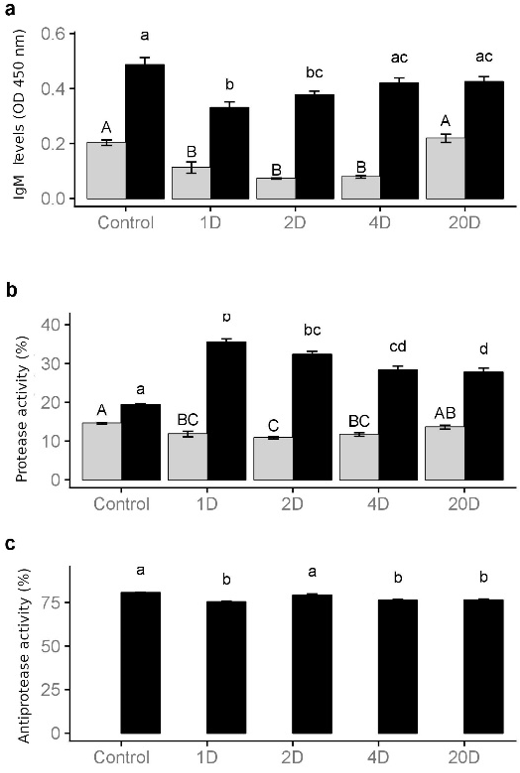
Figure 1: a) Immunoglobulin M (DO 450 nm), b) protease (%) and c) antiprotease (%) activity determined in serum (grey boxes) and mucus (filled boxes) samples of Sparus aurata subjected to different times of feed deprivation: control (fed), 1 day (1D), 2 days (2D), 4 days (4D) and 20 days (20D). Values are means ± S.E. (n=10). The letters above the bars denote the statistical groups with significant differences between the different treatments (P-value < 0.05).
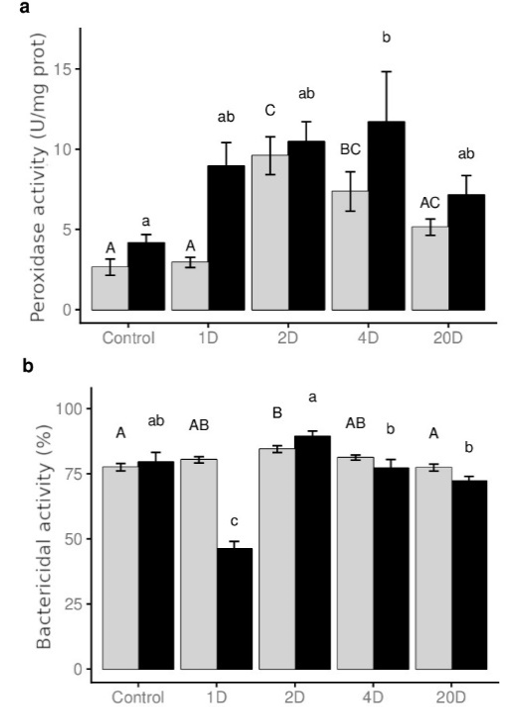
Figure 2: a) Peroxidase activity (U/mg prot) and b) bactericidal activity against Vibrio anguillarum (%) determined in serum (grey boxes) and skin mucus (filled boxes) of Sparus aurata subjected to different times of feed deprivation: control (fed), 1 day (1D), 2 days (2D), 4 days (4D) and 20 days (20D). Values are means ± S.E. (n=10). The letters above the bars denote the statistical groups with significant differences between the different treatments (P value < 0.05).
Conclusion
Several changes have been observed in the studied immune parameters as a consequence of short periods of feed deprivation (< 4 days), and a return to a state of homeostasis perhaps with the aim of trying to maintain cell survival. In general, the activities found in S. aurata skin mucus were in lower proportions than those in serum, except for the bactericidal activity against V. anguillarum, which was similar in serum and skin mucus. This reinforces the assumption that in the mucus there is a large number of antimicrobial peptides (still very little studied), which contribute largely to one of the main functions of mucus as the first external barrier against pathogenic organisms. Decreased IgM concentration and protease activity, as well as increased peroxidase and bactericidal activity in skin mucus could be good indicators of altered nutritional status of fishes, particularly when maintained for short periods of feed deprivation.
Acknowledgments
This work was partly supported by the Spanish Ministry of Economy and Competitiveness (MINECO), co-funded by European Regional Development Funds (Grant no. AGL2017-83370-C3-1-R) and Fundación Séneca de la Región de Murcia (Grupo de Excelencia. Grant no. 19883/GERM/15).
This work was partly supported by the Spanish Ministry of Economy and Competitiveness (MINECO), co-funded by European Regional Development Funds (Grant no. AGL2017-83370-C3-1-R) and Fundación Séneca de la Región de Murcia (Grupo de Excelencia. Grant no. 19883/GERM/15).
References
- Hasan, M.R. (2017). Feeding global aquaculture growth. FAO. Aquaculture Newsletter, 56 II.
- Cerdá, M. J. (2012). Futuro de la alimentación de los peces en granjas marinas. AquaTIC,(37), 78–89.
- Love, R. M. (1980) The chemical biology of fishes, 1 ed.; Eds.; Academic Press Inc., Vol. 2.
- Woo, P. T.; Leatherland, J. F.; Bruno, D. W. (2011). Fish diseases and disorders, 1 ed.; Eds.; CABI, Vol. 3.
- Mohapatra, S.; Chakraborty, T.; Shimizu, S.; Urasaki, S.; Matsubara, T.; Nagahama, Y.; Ohta, K. (2015). Feed deprivation beneficially influences the liver physiology and nutrient metabolism in Edwardsiella tarda infected red sea bream (Pagrus major). Comparative Biochemistry and Physiology Part A: Molecular & Integrative Physiology, 189, 1–10.
- Plisetskaya, E. (1980). Fatty acid levels in blood of cyclostomes and fish. Environmental Biology of Fishes, 5(3), 273–290.
- Sheridan, M. A., Mommsen, T. P. (1991). Effects of nutritional state on in vivo lipid and carbohydrate metabolism of coho salmon, Oncorhynchus kisutch. General and Comparative Endocrinology,81(3), 473–483.
- >Soeters, P. B. (2015). Macronutrient metabolism in feed deprivation and stress. In R.F.; M.; B.R.; R.; and P.B.; S.; (eds.), The Importance of Nutrition as an Integral Part of Disease Management 2015, Vol. 82, pp. 17–25 Nestlé Nutr Inst Workshop Ser. Nestec Ltd. Karger Publishers.
- Folmar, L. C.; Moody, T.; Bonomelli, S.; Gibson, J. (1992). Annual cycle of blood chemistry parameters in striped mullet (Mugil cephalus L.) and pinfish (Lagodon rhomboides L.) fromthe Gulf of Mexico. Journal of Fish Biology 1992,41(6), 999–1011.
- Sandnes, K.; Lie, O.; Waagbo, R. (1988). Normal ranges of some blood chemistry parameters in adult farmed Atlantic salmon, Salmo salar. Journal of Fish Biology, 32(1), 129–136.
- Wedemeyer, Gary A., and Yasutake, W. (1977). Clinical methods for the assessment of the effects of environmental stress on fish health. Department of the Interior, Fish and Wildlife Service, Vol. 89.
- Tietz, N. (1976). Blood gases and electrolytes. Fundamentals of Clinical Chemistry,pp. 919–920.
- Lennox, W. G. (1924). Increase of uric acid in the blood during prolonged feed deprivation. JAMA:The Journal of the American Medical Association, 82(8), 602.
- Izar, G. (1911). Beiträge zur Kenntnis der Harnsäurezerstörung und-bildung. VII. Mitteilung. Hoppe-Seyler’s Zeitschrift für physiologische Chemie, 73(5), 317–334.
- Saglio, P.; Blanc, J. (1989). Intraspecific chemocommunication in immature goldfish, Carassius auratus L.: attraction in olfactometer to free amino acid fractions from skin extracts. Biology of Behaviour, 14, 132–47.
- Rosen, M. W.; Cornford, N. E. (1971). Fluid friction of fish slimes. Nature, 234(5323), 49–51.
- Pickering, A. D. (1974). The distribution of mucous cells in the epidermis of the brown trout Salmo trutta (L.) and the char Salvelinus alpinus (L.). Journal of Fish Biology, 6(2), 111–118.
- Cameron, A. M.; Endean, R. (1973). Epidermal secretions and the evolution of venom glands in fishes. Toxicon, 11(5), 401–410.
- Guardiola, F. A.; Dioguardi, M.; Parisi, M. G.; Trapani, M. R.; Meseguer, J.; Cuesta, A.; Cammarata, M.; Esteban, M. A. (2015). Evaluation of waterborne exposure to heavy metals in innate immune defences present on skin mucus of gilthead seabream (Sparus aurata). Fish & Shellfish Immunology, 45(1), 112–123.
- Guardiola, F. A.; Cuesta, A.; Esteban, M. Á. (2016). Using skin mucus to evaluate stress in gilthead seabream (Sparus aurata L.). Fish & Shellfish Immunology, 59, 323–330.
- Mercado, E. D.; Larrán, A. M.; Pinedo, J.; Tomás-Almenar, C. (2018) Skin mucous: A new approach to assess stress in rainbow trout. Aquaculture, 484, 90–97.
- Khansari, A. R.; Balasch, J. C.; Vallejos-Vidal, E.; Parra, D.; Reyes-López, F. E.; Tort, L. (2018). Comparative immune-and stress-related transcript response induced by air exposure and Vibrio anguillarum bacteria in rainbow trout (Oncorhynchus mykiss) and gilthead seabream (Sparus aurata) mucosal surfaces. Frontiers in immunology, 9.
- Guardiola, F. A.; Saraiva-Fraga, M.; Cuesta, A.; Esteban, M. A. (2018). Changes in natural haemolytic complement activity induced by stress in gilthead seabream (Sparus aurata L.). Fish & Shellfish Immunology, 78, 317–321.
- Palaksha, K.; Shin, G.-W.; Kim, Y.-R.; Jung, T.-S. (2008). Evaluation of non-specific immune components from the skin mucus of olive flounder (Paralichthys olivaceus). Fish & Shellfish Immunology, 24(4), 479–488.
- Bradford, M. M. (1976). A rapid and sensitive method for the quantitation of microgram quantities of protein utilizing the principle of protein-dye binding. Analytical Biochemistry, 72(1-2), 248–254.
- Guardiola, F. A.; Cuesta, A.; Arizcun, M.; Meseguer, J.; Esteban, M. A. (2014). Comparative skin mucus and serum humoral defence mechanisms in the teleost gilthead seabream (Sparus aurata). Fish & Shellfish Immunology, 36(2), 545–551.
- Hanif, A.; Bakopoulos, V.; Dimitriadis, G. (2004). Maternal transfer of humoral specific and non-specific immune parameters to sea bream (Sparus aurata) larvae. Fish & Shellfish Immunology, 17(5), 411–435.
- Quade, M. J.; Roth, J. A. (1997). A rapid, direct assay to measure degranulation of bovine neutrophil primary granules. Veterinary Immunology and Immunopathology, 58(3-4), 239–248.
- Sunyer, J. O.; Tort, L. (1995). Natural hemolytic and bactericidal activities of sea bream Sparus aurata serum are affected by the alternative complement pathway. Veterinary Immunology and Immunopathology, 45(3-4), 333–345.
- Iwama, G. K. (1997). Fish stress and health in aquaculture. Cambridge University Press, Cambridge [England] ; New York.
- Schreck, C. B. (2000). Accumulation and long-term effects of stress in fish. The biology of animal stress, 147-158.
- Carmichael, G. J.; Williamson, J. H.; Woodward, C. A. C.; Tomasso, J. R. (1988). Communications: Responses of northern, Florida, and hybrid largemouth bass to low temperature and low dissolved oxygen. The Progressive Fish?Culturist, 50(4), 225-231.
- Carmichael, G. J.; Tomasso, J. R.; Simco, B. A.; Davis, K. B. (1984). Characterization and alleviation of stress associated with hauling largemouth bass. Transactions of the American Fisheries Society, 113(6), 778-785.
- Vijayan, M. M.; Leatherland, J. F. (1988). Effect of stocking density on the growth and stress-response in brook charr, Salvelinus fontinalis. Aquaculture, 75(1-2), 159-170.
- Gilham, I. D.; Baker, B. I. (1958). A black background facilitates the response to stress in teleosts. Journal of Endocrinology, 105(1), 99-105.
- Barton, B. A.; Schreck, C. B.; Fowler, L. G. (1988). Fasting and diet content affect stress?induced changes in plasma glucose and cortisol in juvenile chinook salmon. The Progressive Fish?Culturist, 50(1), 16-22.
- Vijayan, M. M.; Moon, T. W. (1992). Acute handling stress alters hepatic glycogen metabolism in food-deprived rainbow trout (Oncorhynchus mykiss). Canadian Journal of Fisheries and Aquatic Sciences, 49(11), 2260-2266.
- Pickering, A. D.; Pottinger, T. G. (1989). Stress responses and disease resistance in salmonid fish: effects of chronic elevation of plasma cortisol. Fish physiology and biochemistry, 7(1-6), 253-258.
- Kane, A. S.; Salierno, J. D.; Gipson, G. T.; Molteno, T. C.; Hunter, C. (2004). A video-based movement analysis system to quantify behavioural stress responses of fish. Water Research, 38(18), 3993-4001.
- Wendelaar Bonga, S. E. (1997). The stress response in fish. Physiological reviews, 77(3), 591-625.
- Barton, B. A. (2002). Stress in fishes: a diversity of responses with particular reference to changes in circulating corticosteroids. Integrative and comparative biology, 42(3), 517-525.
- Ryan, J. A.; Hightower, L. E. (1994). Evaluation of heavy?metal ion toxicity in fish cells using a combined stress protein and cytotoxicity assay. Environmental Toxicology and Chemistry, 13(8), 1231-1240.
- Dutta, S.; Chowdhary, G.; Kumar, P.; Mukhopadhay, K.; Narang, A. (2005). Ciprofloxacin administration to very low birth weight babies has no effect on linear growth in infancy. Journal of tropical pediatrics, 52(2), 103-106.
- Hosoya, S.; Johnson, S. C.; Iwama, G. K.; Gamperl, A. K.; Afonso, L. O. B. (2007). Changes in free and total plasma cortisol levels in juvenile haddock (Melanogrammus aeglefinus) exposed to long-term handling stress. Comparative Biochemistry and Physiology Part A: Molecular & Integrative Physiology, 146(1), 78-86.
- Acerete, L.; Balasch, J. C.; Espinosa, E.; Josa, A.; Tort, L. (2004). Physiological responses in Eurasian perch (Perca fluviatilis, L.) subjected to stress by transport and handling. Aquaculture, 237(1-4), 167-178.
- Barton, B. A. (2002). Stress in fishes: a diversity of responses with particular reference to changes in circulating corticosteroids. Integrative and comparative biology, 42(3), 517-525.
- Iwama, G. K.; Afonso, L. O.; Todgham, A.; Ackerman, P.; Nakano, K. (2004). Are hsps suitable for indicating stressed states in fish? Journal of Experimental Biology, 207(1), 15-19.
- Olsen, R. E.; Sundell, K.; Ringø, E.; Myklebust, R.; Hemre, G. I.; Hansen, T.; Karlsen, Ø. (2008). The acute stress response in fed and food deprived Atlantic cod, Gadus morhua L. Aquaculture, 280(1-4), 232-241.
- Trenzado, C. E.; Morales, A. E.; de la Higuera, M. (2008). Physiological changes in rainbow trout held under crowded conditions and fed diets with different levels of vitamins E and C and highly unsaturated fatty acids (HUFA). Aquaculture, 277(3-4), 293-302.
- Choi, C. Y.; Min, B. H.; Jo, P. G.; Chang, Y. J. (2007). Molecular cloning of PEPCK and stress response of black porgy (Acanthopagrus schlegeli) to increased temperature in freshwater and seawater. General and comparative endocrinology, 152(1), 47-53.
- Kaneko, G.; Shirakami, H.; Yamada, T.; ichiro Ide, S.; Haga, Y.; Satoh, S.; Ushio, H. (2016). Short-term fasting increases skeletal muscle lipid content in association with enhanced mRNA levels of lipoprotein lipase 1 in lean juvenile red seabream (Pagrus major). Aquaculture, 452.
- Liao, K.; Meng, R.; Ran, Z.; Cheng, G.; Wang, Y.; Xu, J.; Xu, S.; Yan, X. (2017). Short- term feed deprivation in silver pomfret (Pampus argenteus): molecular effects on lipid mobilization and utilization. Aquaculture Research, 48(9), 4874–4885.
- Heming, T. A.; Paleczny, E. J. (1987). Compositional changes in skin mucus and blood serum during feed deprivation of trout. Aquaculture, 66(3-4), 265–273.
- Setchell, K.; Kritchevsky, D.; Nair, P. (2012). The Bile Acids: Chemistry, Physiology, and Metabolism, 1 ed.; Eds.; Springer US, US, , Volume 4: Methods and Applications.
- Barnes, S.; Gallo, G. A.; Trash, D. B.; Morris, J. S. (1975). Diagnositic value of serum bile acid estimations in liver disease. Journal of Clinical Pathology, 28(6), 506–509.
- Moyes, C. D.; Schulte, P. M. (2007). Principios de fisiología animal, 1 ed.; Eds.; Pearson Addison Wesley.
- Kardong, K. (2007). Vertebrados: anatomía comparada, función y evolución, 4th ed.; Eds.; McGraw-Hill Interamericana de España S.L., España.
- Cox, M. M.; Nelson, D. L. (2014). Lehninger: principios de bioquímica, 6th ed.; Eds.; Omega, S.A.
- Murphy, K.; Travers, P.; Walport, M. (2008). Inmunobiología de Janeway, 7th ed.; Eds.; McGraw-Hill Interamericana.
- Ellis, A. (1999). Immunity to bacteria in fish. Fish & Shellfish Immunology, 9(4), 291–308.
- Harrell, L. W.; Etlinger, H. M.; Hodgins, H. O. (1976). Humoral factors important in resistance of salmonid fish to bacterial disease. II. Anti-Vibrio anguillarum activity in mucus and observations on complement. Aquaculture, 7(4), 363–370.
- Fletcher, T. C.; Grant, P. T. (1969). Immunoglobulins in the serum and mucus of the plaice (Pleuronectes platessa). Biochemical Journal, 115(5), 65.
Citation: Nora Albaladejo-Riad, Cristóbal Espinosa-Ruíz and María Ángeles Esteban. (2020). Feed Deprivation effects on Bactericidal an Immunological activity of Blood and skin mucus, and on blood Chemistry of Gilthead Seabream (Sparus aurata). Journal of Agriculture and Aquaculture 2(1).
DOI: 10.5281/zenodo.3906149
DOI: 10.5281/zenodo.3906149
Copyright: © 2020 María Ángeles Esteban. This is an open-access article distributed under the terms of the Creative Commons Attribution License, which permits unrestricted use, distribution, and reproduction in any medium, provided the original author and source are credited.