Research Article
Volume 2 Issue 1 - 2020
Extracellular Matrix Regenerative Treatment for Cartilage Repair
1Histogen, Inc. 10655 Sorrento Valley Road, San Diego, CA 92121, USA
2Department of Orthopedic Surgery, Northwell Health System, 350 Community Drive, Manhasset, NY 11030, USA
3Synthasome, Inc. 3030 Bunker Hill Street, San Diego, CA 92019, USA
2Department of Orthopedic Surgery, Northwell Health System, 350 Community Drive, Manhasset, NY 11030, USA
3Synthasome, Inc. 3030 Bunker Hill Street, San Diego, CA 92019, USA
*Corresponding Author: Gail Naughton, Histogen, Inc. 10655 Sorrento Valley Road, San Diego, CA 92121, USA.
Received: March 03, 2020; Published: April 09, 2020
Abstract
Articular cartilage lesions caused by traumatic or age-dependent injuries are a major burden to patients and the healthcare system, often requiring painful surgical procedures and long convalescence periods. We have developed a human extracellular matrix-based treatment that leverages the proteins and glycosaminoglycans secreted by hypoxia-induced multipotent cells to create a biological scaffold for the repair and regeneration of hyaline cartilage. Using three established animal models of articular cartilage injury, we evaluated if the human extracellular matrix formulation applied to experimentally induced osteochondral defects was able to improve the healing process as evidenced by visual inspection and histological evaluation. While untreated control groups showed defects characterized by fibrous tissue and suboptimal bone and cartilage regeneration, the animal subjects including rats, rabbits and goats that received the human-derived extracellular matrix treatment induced pronounced tissue regeneration of vascularized subchondral bone and an overlying proteoglycan-rich articular cartilage layer at the site of the defects. The results reported here demonstrate that the bioengineered human extracellular matrix functions as a potential human-source, chondroconducive matrix that supports bone and hyaline articular cartilage regeneration for the use in clinical orthopedic applications.
Keywords: Extracellular Matrix; Regeneration; Hyaline Cartilage
Introduction
Lesions in articular cartilage can cause considerable musculoskeletal morbidity. In the United States alone the clinical burden of cartilage defects and osteoarthritis affects one in five Americans and costs more than $128 billion dollars per year in work losses, outpatient physician visits, and surgeries. [1] Rates of cartilage defects and osteoarthritis are higher and the affected ages are lower in active military service members and veterans than in civilian populations. [2] While there are a number of surgical options, disadvantages of present treatments include highly uneven repair, mixed reparative tissue quality and restriction in the size of defects capable of being treated. We are proposing a human clinical study focused on the clinical development of a human extracellular matrix-based treatment that leverages the proteins and glycosaminoglycans secreted by hypoxia-induced stem cells to create a consistent and safe scaffold for the repair and regeneration of cartilage.
We have developed a technology based on the culture expansion of neonatal human fibroblasts in a closed bioreactor system under suspension and hypoxic conditions to mimic the embryonic environment. During the culture process, the fibroblasts revert to multipotent stem cells, as evidenced by the up-regulation of pluripotent stem cell-associated genes that include, SOX2, OCT4/POU5F1, NANOG and KLF4, and by the expression of stem cell-associated proteins, including nodal, brachyury, nestin and Oct4. [3] The insoluble human extracellular matrix harvested at the end of the manufacturing process is comprised of matrix components with potential to support the infiltration of autologous mesenchymal stem cells and lead to the repair of cartilage.
Hypoxia-induced multipotent stem cells secrete a human extracellular matrix (hECM) that contain components associated with stem cell niches in the body and scarless healing of fetal skin including laminins, osteonectin, decorin, hyaluronic acid, collagen type IV, SPARC, CXCL12, NID1, NID2, NOTCH2, tenascin, thrombospondin, fibronectin, versican, and fibrillin-2. This material can be manufactured reproducibly in closed bioreactor systems under GMP conditions and has been shown to support proliferation of human embryonic stem cells (hESCs) and mesenchymal stem cells (MSCs) and in vitro studies have demonstrated that, unlike other collagen and matrix protein products, hECM does not stimulate human dendritic cell activation, supporting the positive attribute of not stimulating an immune response in vivo.
In vitro studies demonstrated that the ECM supports the migration and proliferation of mesenchymal stem cells, as well as the upregulation of aggrecan and collagen II. [4] These are all important components in cartilage regeneration. Given the results on the in vitro effects on MSC growth and differentiation, we evaluated the hECM to regenerate critical sized osteochondral defects in several small and large animal studies performed at independent facilities. The results reported here demonstrate that the hECM is a potential chondro-condusive matrix that supports bone and hyaline articular cartilage regeneration in osteochondral defects.
Materials and Methods
Preparation and characterization of hECM
The hECM was generated by culturing human neonatal fibroblasts on microcarrier suspension beads in a controlled bioreactor system as previously described. [3,4] Briefly, human neonatal fibroblasts were isolated from foreskin tissue obtained with consent from routine circumcision by enzymatic and mechanical dissociation. The cells were expanded in monolayer culture to produce a frozen master cell bank using DMEM (Invitrogen, Carlsbad, CA, USA) supplemented with 10% fetal bovine serum (SeraCare Life Sciences, Milford, MA, USA) and 1% L-glutamine (Invitrogen, Carlsbad, CA, USA) under 5% CO2 at 37°C. The master cell bank was tested for all known pathogens and virus as established by CBER’s Points to Consider. [5,6]
The hECM was generated by culturing human neonatal fibroblasts on microcarrier suspension beads in a controlled bioreactor system as previously described. [3,4] Briefly, human neonatal fibroblasts were isolated from foreskin tissue obtained with consent from routine circumcision by enzymatic and mechanical dissociation. The cells were expanded in monolayer culture to produce a frozen master cell bank using DMEM (Invitrogen, Carlsbad, CA, USA) supplemented with 10% fetal bovine serum (SeraCare Life Sciences, Milford, MA, USA) and 1% L-glutamine (Invitrogen, Carlsbad, CA, USA) under 5% CO2 at 37°C. The master cell bank was tested for all known pathogens and virus as established by CBER’s Points to Consider. [5,6]
For large scale production, the cells were cultured in a 10-liter working volume, closed-system production bioreactor (Applikon Biotechnology, Delft, The Netherlands) on dextran microcarrier beads (G.E. Healthcare Life Sciences, Marlborough, MA, USA) under hypoxic conditions (3-5% O2) as described.3 A proprietary serum-free cell culture medium consisting of DMEM (Hyclone, Logan, UT, USA) supplemented with rice-derived recombinant human serum albumin (Healthgen Biotechnology Corp., Wuhan, China) was used in the bioreactor system. At the end of the 3-month culture period, the insoluble material consisting of microcarrier beads, cells and deposited ECM was collected, washed in sterile distilled water, and frozen at -80°C.
The frozen insoluble material was thawed, washed twice in sterile PBS (Gibco, Grand Island, NY, USA) and mechanically homogenized (Polytron Kinematica, Luzern, Switzerland) and incubated with sterile-filtered dextranase (Sigma-Aldrich, St. Louis, MO, USA) at 37°C to digest the microcarrier beads.4 The solution was extensively washed with PBS to generate a final hECM material with a paste-like consistency and stored at 4°C until used in experiments.
The hECM material was biochemically characterized by measuring the collagen and sulfated glycosaminoglycan (sGAG) content. [4] The final hECM material was frozen at -80°C and lyophilized in a FreeZone 4.5 Liter benchtop freeze dry system (LabConco, Kansas City MO) to obtain dried material for collagen and sGAG analysis. The hydroxyproline content of lyophilized hECM was determined by first treating lyophilized hECM material with 6 N HCl and heated overnight at 115°C. The samples were neutralized to pH 7-8 with 1 N NaOH and then oxidized with Chloramine-T solution at room temperature followed by the addition of a perchloric acid solution. The samples were then reacted with p-dimethylaminobenzaldehyde (pDAB) and the colorimetric change was measured by at reading at 561 nm absorbance wavelength using a SpectraMax M3 plate reader (Molecular Devices, Sunnyvale, CA). The samples were fitted to a standard curve of 4-hydroxy-L-proline and the collagen content was calculated using a hydroxyproline concentration of 13.5% and expressed as mg collagen/mg dry weight of ECM. [7]
The sGAG content of lyophilized hECM was determined by first enzymatically digesting the material overnight at 60°C in a papain solution containing 0.1 M sodium phosphate, 10 mM EDTA solution, 5 mM cysteine solution, pH 6.5. The samples were reacted with the metachromatic dye 1,9-dimethylmethylene blue (DMB) in a microtiter plate and read at an absorbance wavelength of 525 nm using a SpectraMax M3 plate reader (Molecular Devices, Sunnyvale, CA). The sample values were fitted to a standard curve of chondroitin sulfate from bovine cartilage ranging and expressed as ug sGAG/mg dry weight of ECM. All chemicals for hydroxyproline and sGAG assays were from obtained from Sigma-Aldrich (St. Louis, MO).
In Vitro Induced Gene Expression Studies
Human mesenchymal stem cells from bone marrow or chondrocytes were plated into 6 well plates and cultured to subconfluence in low glucose DMEM supplemented with 5% fetal calf serum and 1% antibiotics. Wells were filled with standard media or media supplemented with hECM. At days 1,3,5,7 cultures were treated to isolate RNA and processed for quantitative PCR.
Human mesenchymal stem cells from bone marrow or chondrocytes were plated into 6 well plates and cultured to subconfluence in low glucose DMEM supplemented with 5% fetal calf serum and 1% antibiotics. Wells were filled with standard media or media supplemented with hECM. At days 1,3,5,7 cultures were treated to isolate RNA and processed for quantitative PCR.
Animal Models
Rat: All small animal studies involving rat and rabbit subjects were performed in accordance with IACUC-approved protocols by trained individuals. The trochlear groove in 20 skeletally mature male Sprague-Dawley rats was exposed and a ~1.7 mm diameter defect was created down to the subchondral plate bilaterally using a drill (MicroAire) with specialized burr. [8] The control group (n=10) was left untreated and the test group (n=10) defects were filled with extracellular matrix that was grouted flush into the defect. Rats were sacrificed at 6 weeks post-operative, the distal femora were removed, fixed and prepared for histology.
Rat: All small animal studies involving rat and rabbit subjects were performed in accordance with IACUC-approved protocols by trained individuals. The trochlear groove in 20 skeletally mature male Sprague-Dawley rats was exposed and a ~1.7 mm diameter defect was created down to the subchondral plate bilaterally using a drill (MicroAire) with specialized burr. [8] The control group (n=10) was left untreated and the test group (n=10) defects were filled with extracellular matrix that was grouted flush into the defect. Rats were sacrificed at 6 weeks post-operative, the distal femora were removed, fixed and prepared for histology.
Rabbit: The articular surface of the distal femur was exposed in 24 skeletally mature male New Zealand White rabbits. Using sterile technique, a 4 mm diameter osteochondral defect to the depth of the subchondral plate was created using a drill (MicroAire) with specialized burr to allow marrow and bloody infiltration, which stimulates microfracture chondroplasty. [9] In the treatment group (n=12), extracellular matrix was filled into the defect via pipette and the surface was smoothed with a blunt periosteal elevator to obtain a smooth congruent surface. The wound was closed in layers with 4-0 vicryl sutures. Animals were sacrificed at 4 and 24 weeks post-treatment (n = 6 per treatment group for each time point) and the femora were removed, fixed and prepared for histology.
Goat: Thirty two (n=32) skeletally mature female goats were part of this study, 16 of which received the hECM treatment article and 16 of which received no treatment. This IACUC-approved study was performed in a preclinical surgical research laboratory of a veterinary college by board-certified veterinary surgeons. Following induction of anesthesia, a medial parapatellar arthrotomy was performed and the articular surface of the medial femoral condyle and the medial trochlea were exposed. [10]
Osteochondral defects of approximately 6.5 mm in diameter and 7.0 mm in depth were created perpendicular to the articular surface in the medial femoral condyle of the right hindlimb using a Mosaicplasty surgical instrumentation system (Smith and Nephew, Inc). The defect depths were measured and approximately 0.4 cc of hECM test article was inserted into the defect using a blunt tipped 18G needle until flush with the articular surface. Half of the test and control animals were sacrificed at 4 months and the remainder at 12 months (n = 8 per treatment group for each time point). Goats were euthanized by intravenous barbiturate overdose according to the 2013 AVMA guidelines for the euthanasia of animals. A gross necropsy was performed and both the right and left hindlimbs were harvested and stored on ice for approximately one day prior to subsequent analysis. The knee joint was further dissected to obtain the distal femur containing the site of the defect. Photographs were taken of the defect site and the tissue containing the defect was prepared for histology.
Results
Characterization
The bioengineering production process results in hECM material that is consistent in collagen and glycosaminoglycan (sGAG) composition (Figure 1). Proteomic mass spectrometry analysis showed that the hECM contained a variety of collagens and other matrix proteins that are associated with embryonic and adult healthy tissue. The most prominent components are listed in Table 1, ranked by relative abundance.
The bioengineering production process results in hECM material that is consistent in collagen and glycosaminoglycan (sGAG) composition (Figure 1). Proteomic mass spectrometry analysis showed that the hECM contained a variety of collagens and other matrix proteins that are associated with embryonic and adult healthy tissue. The most prominent components are listed in Table 1, ranked by relative abundance.
Protein Description |
Collagen alpha-1(I) chain |
Collagen alpha-2(I) chain |
Collagen alpha-3(VI) chain |
Collagen alpha-1(VI) chain |
Tenascin |
Actin, cytoplasmic 2 |
Vimentin |
Isoform 17 of Fibronectin |
Collagen alpha-1(XII) chain |
Decorin |
Fibrillin-1 |
Collagen alpha-1(III) chain |
Collagen alpha-2(V) chain |
Transforming growth factor-beta-induced protein ig-h3 |
Collagen alpha-2(VI) chain |
Isoform 2 of Tubulin alpha-4A chain |
Collagen alpha-1(V) chain |
Glia-derived nexin |
Basement membrane-specific heparan sulfate proteoglycan core protein |
Table 1: Summary of major protein components in hECM as determined by mass spectrometric analysis.
In Vitro Induced Gene Expression Analysis
Both MSCs and chondrocytes in hECM treated cultures showed a >3 fold [P < 0.05) down regulation of collagen I and chondrocytes demonstrated a 5-fold (P<0.03) increase of aggrecan gene expression by day 5 (Figure 2). MSCs cultured for 7 days in the presence of hECM showed an intense positive safranin-O staining as compared to the control cultures thus demonstrating proteoglycan secretion in the treated cultures (Figure 3).
Both MSCs and chondrocytes in hECM treated cultures showed a >3 fold [P < 0.05) down regulation of collagen I and chondrocytes demonstrated a 5-fold (P<0.03) increase of aggrecan gene expression by day 5 (Figure 2). MSCs cultured for 7 days in the presence of hECM showed an intense positive safranin-O staining as compared to the control cultures thus demonstrating proteoglycan secretion in the treated cultures (Figure 3).
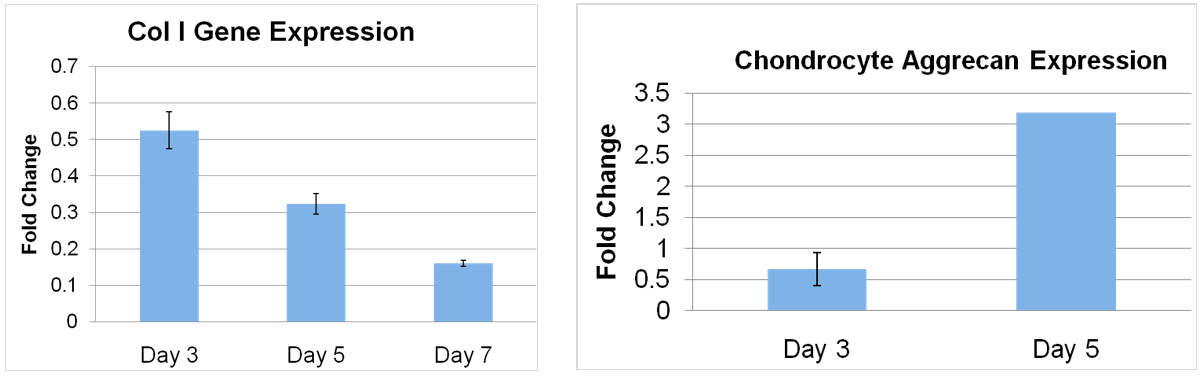
Figure 2: (Left) Collagen I gene expression. (Right) Aggrecan expression. Human MSCs or passaged chondrocytes were plated into 6 well plates and cultured to subconfluence in DMEM. Wells were filled with standard media (control) or media supplemented with hECM. At days 1,3,5 and 7 cultures were treated to isolate RNA and processed for quantitative RT-PCR.
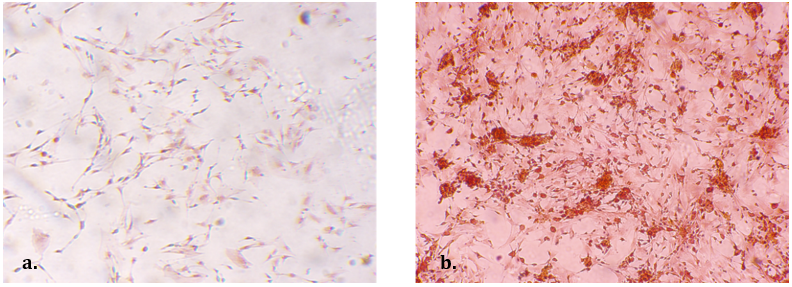
Figure 3: (Left) Control MSC cultures 7 days in vitro. (Right) hECM supplemented cultures. Note the intense positive safranin-O staining in the hECM cultures compared to control. (200x orig. mag)
Macroscopic analysis of the femurs in the rats showed evidence of the remaining defect in untreated animals while sites treated with hECM showed smooth cartilage healing (Figure 4). The quality of repair was analyzed histologically with safranin-O/fast green staining and quantified by a blinded observer using the Modified O’Driscoll Score. [11] Results of the control group showed a defect site containing extensive fibrous tissue, less mature osteochondral regeneration and without restoration of the articular cartilage layer. The treated group showed congruent and smooth cartilage regeneration, viable matrix-producing chondrocytes and integration with the surrounding cartilage and well vascularized bone up to the tide mark. Safranin O staining revealed deposition of glycosaminoglycan matrix in the regenerated articular cartilage tissue layer of treated defects, whereas staining was absent in the untreated defects. The study was completed in duplicate, with both studies resulting in statistically significant improvements in articular cartilage formation in the treated versus untreated groups as evaluated by the Modified O’Driscoll scoring system (Student’s two-tailed t-test, p < 0.001). These findings on small defects support the hypothesis that the extracellular matrix promotes improvement in cartilaginous repair.
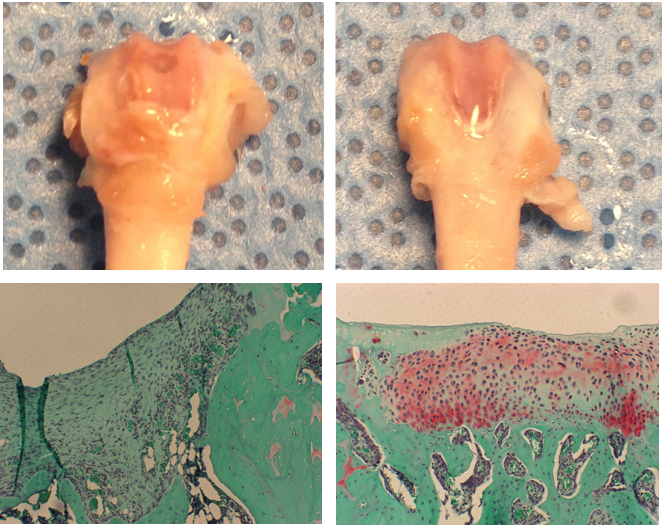
Figure 4: Rats were sacrificed at 6 weeks post-operative, the distal femora were removed, fixed and prepared for histology. (Left) Untreated control. (Right) Treated with hECM. (Top) Macroscopic analysis of the femurs in the rats showed evidence of the remaining defect in untreated animals, while sites treated with hECM showed smooth cartilage healing. (Bottom) Repair quality was analyzed histologically with safranin-O/fast green staining and quantified by a blinded observer using the modified O’Driscoll Score (student’s two-tailed t-test, p < 0.001).
In the rabbit model, the quality of repair was analyzed histologically with safranin-O/fast green staining and graded by a blinded observer using the Modified O’Driscoll Scoring system (Figure 5). Histological results of the untreated group showed repair with a fibrous tissue that did not restore the articular surface. Results show that the hECM-treated group demonstrated a greater degree of articular cartilage formation characterized by increased structural integrity and homogeneity, proteoglycan-rich chondrocyte cellularity and clustering, and subchondral bone reconstruction. There was also a decrease in degenerative changes noted in the adjacent cartilage layer than compared to the untreated defect, which was characterized by degenerative articular cartilage tissue surrounding the defect site. These findings confirm our hypothesis that extracellular matrix promotes the improvement of cartilaginous repair in microfracture procedures.
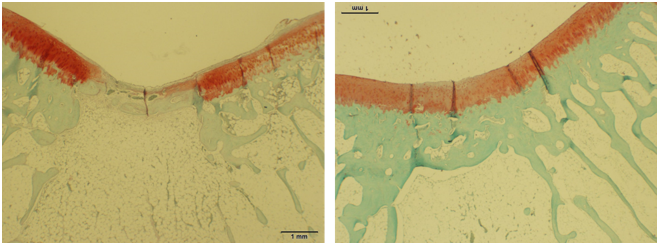
Figure 5: After induction and treatment, rabbit wounds were closed in layers with 4-0 vicryl sutures. Animals were sacrificed at 4- and 24-weeks post-treatment and the femora were removed, fixed and prepared for histology. (Left) Untreated. (Right) Treated. The quality of repair was analyzed histologically with safranin-O/ fast green staining and graded by a blinded observer using the Modified O’Driscoll Scoring system.
Following the positive results observed in the small animal models of osteochondral defects, it was hypothesized that treatment of a larger osteochondral defect with hECM can improve the quality of the resulting tissue repair and regeneration in larger mammals, such as the goat. Macroscopic observation showed the extracellular matrix-treated defect exhibited substantial filling of the defect with a tissue resembling hyaline articular cartilage. The untreated, control defects displayed deep defects with very little closure and evidence of erosion and collapse at the margins. Histologically, the 4-month untreated, control animals showed no bone remodeling or cartilage growth in the defect area while treated animals showed good remodeling and new hyaline cartilage formation across and into the defect. All hECM-treated animals continued to improve over 12 months with more mature hyaline cartilage and bone integrating with the adjacent normal tissue observed at the histological level. The untreated defects at 12 months were characterized by large voids filled with fibrous tissue and demonstrating insignificant evidence of subchondral bone and articular cartilage tissue regeneration (Figure 6).
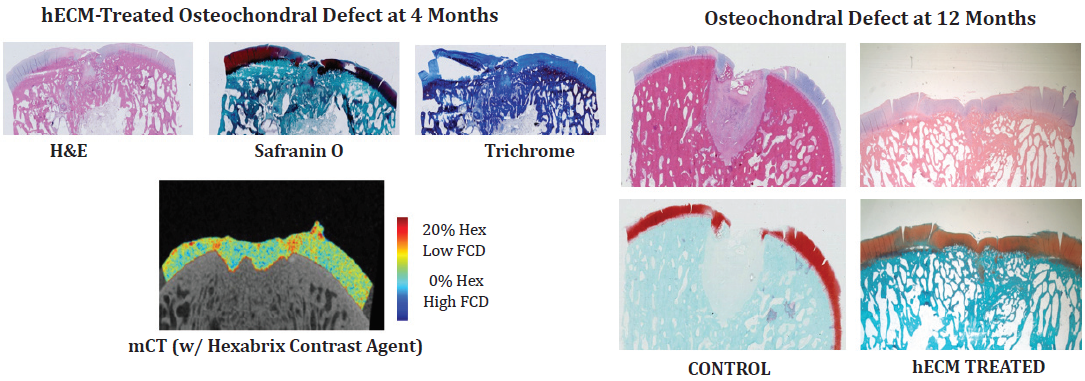
Figure 6: Half of the test and control animals were sacrificed at 4 months and the remainder at 12 months. A gross necropsy was performed and both the right and left hindlimbs were harvested and stored on ice for approximately one day prior to subsequent analysis. The knee joint was further dissected to obtain the distal femur containing the site of the defect.
Conclusion
The results from these series of in vivo studies demonstrate that hECM produced by multipotent cells grown under hypoxic conditions can provide an improved milieu to support the ingrowth of autologous MSCs and the regeneration of hyaline cartilage and mature bone following a critical-sized osteochondral defect. The embryonic-like naturally secreted human biomaterial scaffold stimulates native stem cell infiltration and differentiation, upregulates the cells to produce aggrecan and collagen type 2, and results in hyaline cartilage generation.
Articular cartilage and mature bone repair remain a challenge for orthopedic surgeons. The use of biomaterial scaffolds that support the infiltration of native stem cells and the regeneration of cartilage and bone are a logical translational step toward improving the quality of cartilage repair.
References
- Mujeeb, A., Ge, Z. (2014). Biomaterials for cartilage regeneration. J Am Acad Ortho Surg 22: 1.
- Diduch, D.R., Insall, J.N., Scott, W.N., Scuderi, G.R., Font-Rodriguez, D. (1997). Total knee replacement in young, active patients: Long-term follow-up and functional outcome. J Bone Joint Surg Am 79(4): 575.
- Pinney, E., Zimber, M., Schenone, A., Montes-Camacho, M., Ziegler, F., Naughton, G. K. (2011). Human Embryonic-like ECM (hECM) stimulates proliferation and differentiation in stem cells while killing cancer cells. Intern J Stem Cells 4: 70.
- Zhou, Y., Zimber, M., Yuan, H., Naughton, G.K., Fernan, R., Li, WJ. (2016). Effects of Human Fibroblast-Derived Extracellular Matrix on Mesenchymal Stem Cells. Stem Cell Rev and Rep 12(5): 560.
- Kruse, P. F. Jr, Patterson, M. K. Jr (Eds). (1973). Tissue culture methods and applications. Academic Press, New York.
- Administration UFaD. (1993). Points to consider in the characterization of cell line used to produce biologicals. US Department of Health and Human Services, Bethesda, MD.
- Neuman, R. E., Logan, M. A. (1950). The determination of collagen and elastin in tissues. J Bio Chem 186: 549.
- Dahlin, R.L., Kinard, L.A., Lam, J., Needham, C.J., Lu, S., Kasper, F.K., Mikos, A.G. (2014). Articular chondrocytes and mesenchymal stem cells seeded on biodegradable scaffolds for the repair of cartilage in a rat osteochondral defect model. Biomaterials 35(26): 7460.
- Kim, M., Foo, L.F., Uggen, C., Lyman, S., Ryaby, J.T., Moynihan, D.P., Grande, D.A., Potter, H.G., Pleshko, N. (2010). Evaluation of Early Osteochondral Defect Repair in a Rabbit Model Utilizing Fourier Transform–Infrared Imaging Spectroscopy, Magnetic Resonance Imaging, and Quantitative T2 Mapping. Tissue Eng Part C 16(3): 355.
- Chan, E.F., Liu, I.L., Semler, E.J., Aberman, H.M., Simon, T.M., Chen, A.C., Truncale, K.G., Sah, R.L. (2012). Association of 3-Dimensional Cartilage and Bone Structure with Articular Cartilage Properties in and Adjacent to Autologous Osteochondral Grafts after 6 and 12 months in a Goat Model. Cartilage. Jul 1; 255.
- Zalewski, T., Lubiatowski, P., Jaroszewski, J., Szcze?niak, E., Ku?mia, S., Kruczy?ski, J., Jurga S. (2008). Scaffold-aided repair of articular cartilage studied by MRI. MAGMA 21: 177.
Citation: Gail Naughton., et al. (2020). Extracellular Matrix Regenerative Treatment for Cartilage Repair. Journal of Orthopaedic and Trauma Care 2(1). DOI: 10.5281/zenodo.3755543
Copyright: © 2020 Gail Naughton. This is an open-access article distributed under the terms of the Creative Commons Attribution License, which permits unrestricted use, distribution, and reproduction in any medium, provided the original author and source are credited.