Research Article
Volume 2 Issue 1 - 2020
Development of an Acoustic wave Nano Sensor for Hg(II) using Quartz Crystal Microbalance Modified with gold Nanoparticles- 1,6-hexanedithiol
1Department of Chemistry, University of Tripoli, Tripoli, Libya
2General of advanced laboratory of Chemical Analysis, Libyan authority to search, science and technology, Tripoli-Libya
2General of advanced laboratory of Chemical Analysis, Libyan authority to search, science and technology, Tripoli-Libya
*Corresponding Author: Abdunnaser Etorki, Department of Chemistry, University of Tripoli, Tripoli, Libya.
Received: August 05, 2020; Published: August 14, 2020
Abstract
The development of an acoustic wave Nano sensor for the detection of mercury ions from aqueous solutions based on the formation of self-assembled monolayer of 1,6-hexanedithiol (HDT) films on Gold nanoparticles. Gold nanoparticles were deposited on 10 MHz AT-cut Pt- piezoelectric quartz crystal resonators and finally modified with 1,6-hexanedithiol (HDT) monolayers. Measurements of resonant frequency for bare and modified electrodes, both immersed and emersed, were used to determine the extent of the ligand immobilization; since the films are extremely thin, the frequency response can be interpreted in purely gravimetric terms using the Sauerbrey equation. The quality and integrity of the HDT films were assessed by cyclic voltammetric measurements of [Fe (CN)6]-3,-4 redox couple chemistry.
HDT-functionalised electrodes were exposed to solutions of mercury ions in order to assess the ability of the surface-bound ligand to complex the Hg (II) ions. The co-exiting metal ions such as Pb2+, Cd2+, Cu2+, Zn+2, Ni2+ and Ag+ were examined with the same ligand, single Hg(II) ion solutions were used and the extent of Hg(II) complexation was determined (via the QCM frequency change) as a function of concentration. Factors associated with solvent and solvation were explored via parallel sets of measurements under immersed and emersed conditions. The variation of surface concentrations with solution concentration was evaluated using a range of isotherms-Langmuir, Temkin, Freundlich, Frumkin, El-Awady and Flory-Huggins- based on different physical models. Selectivity for different metal ions was assessed by comparing the binding constants derived from the isotherms for single Hg (II) ion solutions and by competitive binding experiments. The combination of (HDT) SAM on Au NPs selectivity and Pt-QCM sensitivity provides a potentially useful Hg (II) ion sensor in the nanomolar range. Supporting XPS measurements provide unequivocal evidence of the presence of surface- bound metal(s), together with metal speciation, Hg: HDT stoichiometry, and selectivity between Hg (II) ions in competitive binding experiments. The detection limit can be determined to be 2x10-8 mol /l, the acoustic wave Nano sensor shows good stability and reproducibility on dry crystals than wet crystals with recovery rate of 97-101%.
Keywords: Acoustic wave nanosensor; Detection of mercury; Self-assembled monolayer; Isotherms; Selectivity; EQCM; XPS; Mole ratio
Introduction
The applications of QCM for acoustic wave mass sensing in liquids has been reviewed [1,2,3]. They reported sensors based on QCM have been applied to metal ion analyses in solution using electrodeposition, adsorption and stripping methods. Analytical procedures for trace metal ions using SAM-modified electrodes with electrochemical determination include electrodes modified by mercaptocaracetic acid for Hg (II) detection [4] and 4-mercapto-n-alkylpyridinium for Cr (VI) detection [5] down to 10-10 M, by 2-mercaptobenzimidazole for Hg (II) determination down to 10-9 M [6], and carbamoylphosphonic acid for Cd (II), Cu (II) and Pb(II) detection. Mercapto compounds are of interest because of their capability to form a stable bond with mercury.
Short chain alkyl thiols with charged or bulky end groups like mercaptoethanesulfonate (MES), mercaptopropanesulfonate (MPS), mercaptoacetic acid (MAA), mercaptopropionic acid (MPA) or aminoethane thiol (AET) lead to disorganized and porous monolayers on the electrode face [7,8]. Longer chains lead to more ordered structures, but self-assembled mono-layers (SAMs) of the less ordered shorter alkyl chain compounds are more useful for analytical applications [9]. The disorder in the film allows the analyte to diffuse through the film. Gold disk electrodes coated with MAA or MES have been used to detect copper [10] and a self- assembled monolayer of DNA on gold was used for the detection of lead [11]. SAMs of different ligands on QCM have been investigated on mercury film electrodes as well as gold electrodes for the determination of cadmium [12,13] and shorter mercaptocarboxylic compounds are thought to provide superior sensitivity.
The concentration measurements of Hg (II) ions in aquatic solutions are of great importance in assessing environmental damage and possible success of remediation efforts. The surface plasmon resonance (SPR) technique was used by Zare et al [14,15] to detect Hg(II) ions using 1,6-hexanedithiol (HDT). Changes in the SPR signal allowed quantification of the concentration of Hg (II) in solution from 1.0 nM to 1.0 mM, this method also allowed selective detection of Hg (II) ions in the presence of interfering ions. The SAMs of HDT modified Au nanoparticles will be used in this paper to adsorb Hg (II) ions using the EQCM as a nano sensor to monitor the associated changes in mass at a HDT SAM modified Au nanoparticles. The mercapto group (-SH) is known to react with heavy metal ions to form mercaptide [16]. These thiol functional groups are known to have very high specificity for binding soft cations such as Hg (II) ions from aqueous solutions than other metal ions to various surfaces [17,18]. Previous studies on HDT using X-ray photoelectron spectroscopy (XPS) [19] and scanning tunneling microscopy (STM) [20] confirmed that the HDT molecules formed SAMs with only one thiol group attached to the gold surface, leaving the other thiol group free to adsorb Hg(II) ions from aqueous solutions [21,22].
In this work a novel Pt-QCM modified with Au nanoparticles an acoustic wave nano sensor for rapid, highly selective for determination of Hg(II) ions was developed. 1,6-hexanedithiol (HDT) was selected a immobilized ligand. AuNPs were firstly deposited on Pt-QCM resonator, Au NPs have a very large surface area, which is favourable for HDT self-assembled monolayers.
Experimental
Reagents
1,6-Hexanedithiol (99.9% Aldrich), Hg(NO3)2 , Cd(NO3)2, Pb(NO3)2, Cu(NO3)2 , Zn(NO3)2 , Ni(NO3)2, Ag(NO3)2 and KNO3 (all Aldrich) were used as received. Solutions for SAM deposition were made using ethanol (99.8%, Merck) and for metal ion binding/assay using ultra-pure water (Millipore Milli-Q).
Reagents
1,6-Hexanedithiol (99.9% Aldrich), Hg(NO3)2 , Cd(NO3)2, Pb(NO3)2, Cu(NO3)2 , Zn(NO3)2 , Ni(NO3)2, Ag(NO3)2 and KNO3 (all Aldrich) were used as received. Solutions for SAM deposition were made using ethanol (99.8%, Merck) and for metal ion binding/assay using ultra-pure water (Millipore Milli-Q).
Preparation of Au NPs surfaces
Pt-QCM electrode was successively rinsed with a fresh Piranha solution (30% H2O2 : 98% H2SO4, 1 : 3 by volume) at room temperature for 1-3 minutes [23] rinsed thoroughly by Millipore Milli-Q deionised water, dried under a stream of nitrogen, rinsed again with absolute ethanol to remove any remaining water and dried under a stream of nitrogen. Then the Pt-QCM electrode was directly linked with Cu wire and immersed into the HAuCl4 solution together. KNO3 was added into as an electrolyte additive. After a 2h time, we took the electrode out and rinsed it with Millipore Milli-Q deionised water. The electrode frequency was measured to determine the deposition amount. The deposition amount and deposition rate electrolyte deposition can be measured by EQCM. The results are shown in Figure 1. It could be seen that the amount of Au nanoparticles was almost proportion with deposition time.
Pt-QCM electrode was successively rinsed with a fresh Piranha solution (30% H2O2 : 98% H2SO4, 1 : 3 by volume) at room temperature for 1-3 minutes [23] rinsed thoroughly by Millipore Milli-Q deionised water, dried under a stream of nitrogen, rinsed again with absolute ethanol to remove any remaining water and dried under a stream of nitrogen. Then the Pt-QCM electrode was directly linked with Cu wire and immersed into the HAuCl4 solution together. KNO3 was added into as an electrolyte additive. After a 2h time, we took the electrode out and rinsed it with Millipore Milli-Q deionised water. The electrode frequency was measured to determine the deposition amount. The deposition amount and deposition rate electrolyte deposition can be measured by EQCM. The results are shown in Figure 1. It could be seen that the amount of Au nanoparticles was almost proportion with deposition time.
Preparation of functionalized SAMs of HDT
1,6-hexanedithiol Self-assembled monolayers were prepared by immersing the Pt-Au NPs quartz crystals in 25ml of 10mM of 1,6-hexanedithiol ethanolic modification solution for 24-48 hours to form a full monolayer on the surface of Au nanoparticles. After the functionalization, the solutions were removed from the QCM cell; the crystals were rinsed with absolute ethanol to remove any excess on the surface and blown dry with nitrogen. All the modifications were done at room temperature.
1,6-hexanedithiol Self-assembled monolayers were prepared by immersing the Pt-Au NPs quartz crystals in 25ml of 10mM of 1,6-hexanedithiol ethanolic modification solution for 24-48 hours to form a full monolayer on the surface of Au nanoparticles. After the functionalization, the solutions were removed from the QCM cell; the crystals were rinsed with absolute ethanol to remove any excess on the surface and blown dry with nitrogen. All the modifications were done at room temperature.
Acoustic wave measurements
Frequency measurements were routinely made relative to a reference crystal and at least once in each series an absolute measurement was made to ensure the direction of frequency change was known unambiguously; in both cases, these measurements were made with All acoustic wave measurements were performed using eQCM 10M electrochemical quartz crystal microbalance (Gamry Instruments, Warminster, PA, USA).
Frequency measurements were routinely made relative to a reference crystal and at least once in each series an absolute measurement was made to ensure the direction of frequency change was known unambiguously; in both cases, these measurements were made with All acoustic wave measurements were performed using eQCM 10M electrochemical quartz crystal microbalance (Gamry Instruments, Warminster, PA, USA).
Electrochemical measurements
All electrochemical measurements were carried out in a standard three-electrode cell configuration. The working electrode was one of the eQCM Pt-coated quartz crystals with an area of 0.21 cm2 (Gamry Instruments). The counter electrode was a Pt gauze. The reference was a saturated calomel electrode (SCE), with respect to which all working electrode potentials were controlled (using Reference 3000 Potentiostat/Galvanostat/ZRA, Gamry Instruments, and Warminster, PA, USA) and are quoted. Appraisal of HDT integrity (see below) was based on cyclic voltammetric responses to 5 mM K3Fe(CN)6/1 M KCl in the range -0.4 < E/V < 0.6V (scan rates, v = 5, 10, 50, 100 mV s-1; T = 20°C).
All electrochemical measurements were carried out in a standard three-electrode cell configuration. The working electrode was one of the eQCM Pt-coated quartz crystals with an area of 0.21 cm2 (Gamry Instruments). The counter electrode was a Pt gauze. The reference was a saturated calomel electrode (SCE), with respect to which all working electrode potentials were controlled (using Reference 3000 Potentiostat/Galvanostat/ZRA, Gamry Instruments, and Warminster, PA, USA) and are quoted. Appraisal of HDT integrity (see below) was based on cyclic voltammetric responses to 5 mM K3Fe(CN)6/1 M KCl in the range -0.4 < E/V < 0.6V (scan rates, v = 5, 10, 50, 100 mV s-1; T = 20°C).
XPS characterization
XPS measurements were made on a Scienta ESCA 300 photoelectron spectrometer at the NCESS Facility, CCLRC Daresbury Laboratory. A rotating anode source (operated at 14 kV and 20 mA) generated monochromated Al Ka radiation (1486.7 eV). Although internal calibration to the Au 4f7/2 line at 84.0 eV was used to compensate for sample charging [24].
XPS measurements were made on a Scienta ESCA 300 photoelectron spectrometer at the NCESS Facility, CCLRC Daresbury Laboratory. A rotating anode source (operated at 14 kV and 20 mA) generated monochromated Al Ka radiation (1486.7 eV). Although internal calibration to the Au 4f7/2 line at 84.0 eV was used to compensate for sample charging [24].
Binding Hg (II) to SAM HDT on Au NPs-Pt-QCM
A stock solution of 1.0 mM of Hg (II) was used to prepare all concentrations of Hg (II) ions. Hg (II) at concentrations from 1.0 nM to 1.0 mM at pH= 5.5 was bound to the surface of 1,6-hexanedithiol (HDT) modified Au nanoparticles. The preconcentration time for low concentrations was about 10 min and at high concentration was about 1-5 min. Every frequency shift measurement for every concentration was repeated four times on the same surface coverage of the HDT on emersed and immersed crystal, to examine reproducibility. The practical work was divided to three stages:
A stock solution of 1.0 mM of Hg (II) was used to prepare all concentrations of Hg (II) ions. Hg (II) at concentrations from 1.0 nM to 1.0 mM at pH= 5.5 was bound to the surface of 1,6-hexanedithiol (HDT) modified Au nanoparticles. The preconcentration time for low concentrations was about 10 min and at high concentration was about 1-5 min. Every frequency shift measurement for every concentration was repeated four times on the same surface coverage of the HDT on emersed and immersed crystal, to examine reproducibility. The practical work was divided to three stages:
Strategy (Stage one)
In stage one, 1,6-hexanedithiol-attached ligands bound to Au nanoparticle surfaces on the Pt-QCM cell produce a mass change of the QCM-SAM-ligand to QCM-SAM-ligand-Hg(II) composite resonator. Hg(II) ion binding to SAMs was determined at pH 5.5 and the following were measured:
In stage one, 1,6-hexanedithiol-attached ligands bound to Au nanoparticle surfaces on the Pt-QCM cell produce a mass change of the QCM-SAM-ligand to QCM-SAM-ligand-Hg(II) composite resonator. Hg(II) ion binding to SAMs was determined at pH 5.5 and the following were measured:
- Frequency change for emersed Pt-AuNPs crystal with 1,6-hexanedithiol (Δfdry-SAM).
- Frequency change for immersed crystal with 1,6-hexanedithiol + 8 ml of deionised water in EQCM cell (Δfwet-SAM).
- Frequency change for immersed crystal with 1,6-hexanedithiol + 8 ml of deionised water + 2ml 1000 ppm of Hg(II) solution (Δfwet-SAM-Hg(II)).
- After drying the crystal in procedure 3, the frequency change for the emersed crystal with 1,6-hexanedithiol- Hg(II) (Δfdry-SAM-Hg(II)).
The following were then calculated:
(1) Δfdry-Hg (II) = Δfdry-SAM-Hg (II) - Δfdry-SAM.
(2) Δfwet-Hg (II) = Δfwet-SAM-Hg (II) - Δfwet-SAM.
Measurements were made at room temperature, typically 20°C.
(1) Δfdry-Hg (II) = Δfdry-SAM-Hg (II) - Δfdry-SAM.
(2) Δfwet-Hg (II) = Δfwet-SAM-Hg (II) - Δfwet-SAM.
Measurements were made at room temperature, typically 20°C.
(Stage two)
In this stage the concentration of Hg (II) ions was changed from 1.0mM to 1000 mM. The frequency shifts were measured for SAM covered dry quartz crystal + 2ml deionised water + 10ml of Hg (II) solution.
In this stage the concentration of Hg (II) ions was changed from 1.0mM to 1000 mM. The frequency shifts were measured for SAM covered dry quartz crystal + 2ml deionised water + 10ml of Hg (II) solution.
Strategy (Stage three)
In this stage, the comparison between the stoichiometry of the Hg (II) ions to HDT from stage one and two will be studied.
In this stage, the comparison between the stoichiometry of the Hg (II) ions to HDT from stage one and two will be studied.
Results and Discussion
Characterization of Au NPs/HDT SAMs
Cyclic voltammetric responses at moderate scan rates for the Fe(CN)63-/4- couple at bare Au were reversible are shown in Figure 2. Deposition of the BDMT SAM resulted in modest departure from this behaviour. Fits to the standard Nicholson-Shain model [25] of the variation with potential scan rate of the peak separation gave an electron transfer rate constant of 3.2 x 10-5 cm s-1. This suggests that the HDT layer is exhibits better blocking properties for the electron transfer and form well denser packed organized monolayers on Au NPs.
Cyclic voltammetric responses at moderate scan rates for the Fe(CN)63-/4- couple at bare Au were reversible are shown in Figure 2. Deposition of the BDMT SAM resulted in modest departure from this behaviour. Fits to the standard Nicholson-Shain model [25] of the variation with potential scan rate of the peak separation gave an electron transfer rate constant of 3.2 x 10-5 cm s-1. This suggests that the HDT layer is exhibits better blocking properties for the electron transfer and form well denser packed organized monolayers on Au NPs.
HDT can be determined by gravimetric interpretation of the frequency shift using the Sauerbrey equation [26]:
Δf = -2.26 x 10-6 f 2Δm / A = C1 Δm (1)
Where Δf is the frequency change corresponding to a mass loading Dm, f is fundamental frequency of the crystal (10 MHz in this study), A is the electrode area (0.23 cm2 in this study) and C1 is used to represent the collection of constants that define the QCM sensitivity. The presentational form of the admittance spectra is not ideal for measuring the frequency shift, but this can be done either by curve fitting or – as was subsequently the case here - by measuring the resonant frequency in a simple eQCM oscillator circuit.
Δf = -2.26 x 10-6 f 2Δm / A = C1 Δm (1)
Where Δf is the frequency change corresponding to a mass loading Dm, f is fundamental frequency of the crystal (10 MHz in this study), A is the electrode area (0.23 cm2 in this study) and C1 is used to represent the collection of constants that define the QCM sensitivity. The presentational form of the admittance spectra is not ideal for measuring the frequency shift, but this can be done either by curve fitting or – as was subsequently the case here - by measuring the resonant frequency in a simple eQCM oscillator circuit.
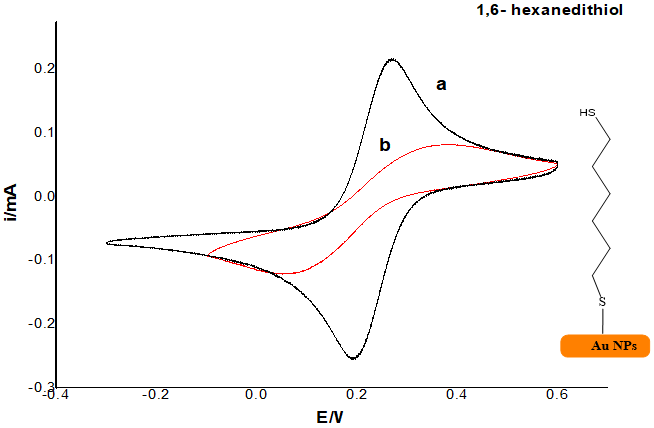
Figure 2: Cyclic voltammograms of gold nanoparticles modified screen printed carbon electrode (a) and (b) modified with 1,6-hexanedithiol in 5 mM K3Fe(CN)6 + 1M KCl. SAM formed in 10 mM thiol ethanol solution, 48 h immersion. Scan rate 0.1V s-1.
SEM images of Pt-QCM electrode and Pt-Au NPS were shown in Figures 3 and 4.
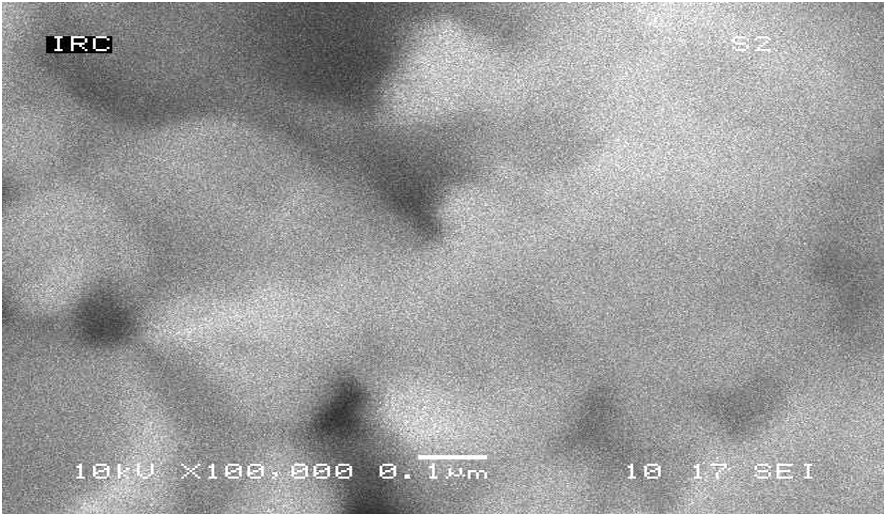
Figure 3: SEM image of Pt-QCM electrode was successively rinsed with a fresh Piranha solution and Milli-Q deionised water.
The binding of Hg (II) to HDT SAM modified Pt-AuNPs was studied. The same ligand was also examined for binding other metal ions such as Pb2+, Cd2+, Cu2+, Zn+2, Ni2+ and Hg2+. The experimental adsorption isotherms were determined for the same coverage of the monolayer with pH=5.0 for Hg (II), pH=4.8 for lead, pH=5.4 for copper, pH=6.5 for cadmium, pH= 5.0 for zinc, pH=6.0 for silver and pH=5.5 for nickel. The range of concentrations for all metal ions was from 1.0nM to 1 mM. The results of adsorption isotherms of Hg2+, Pb2+, Cd2+, Cu2+, Zn+2, Ni2+ and Ag+ to the same ligand on the dry (emersed) and wet (immersed) crystals are shown in Figures 5 and 6).
The frequency shifts were proportional to Hg (II) concentration up to 10 mM, and steady from 50 μM. The saturation surface of Hg (II) ions at 100 μM for dry (emersed) and wet (immersed) crystals was 2.86±0.05 and 3.33± 0.12 nmol cm-2 (n=4), respectively. The surface coverage of the HDT after 18h modification time estimated on emersed and immersed crystal was 2.48 and 2.60 nmol cm-2, respectively. The mole ratio between the saturation surface coverage of Hg (II) ions and HDT was 1.15±0.04 and 1.28±0.06, respectively. The adsorption isotherms show that Hg (II) adsorption isotherms steeply increased with increased concentration of the solution until the plateau state at 1mM of Hg (II). For other metal ions, the adsorption isotherms are less steep. The experimental adsorption data for binding different concentrations of lead ions to the monolayer were fitted to different isotherms such as Langmuir, Temkin, Freundlich, Frumkin, El-Awady and Flory-Hugins isotherms as seen in figures 7 and 8. It was found that the Temkin fits very well to experimental data for all metal ions, based on a high correlation factor (R2) for both dry and wet crystals. From Temkin linear regression models one can estimate the binding constant for each metal ion and compared these binding constants. The results are listed in Table 1.
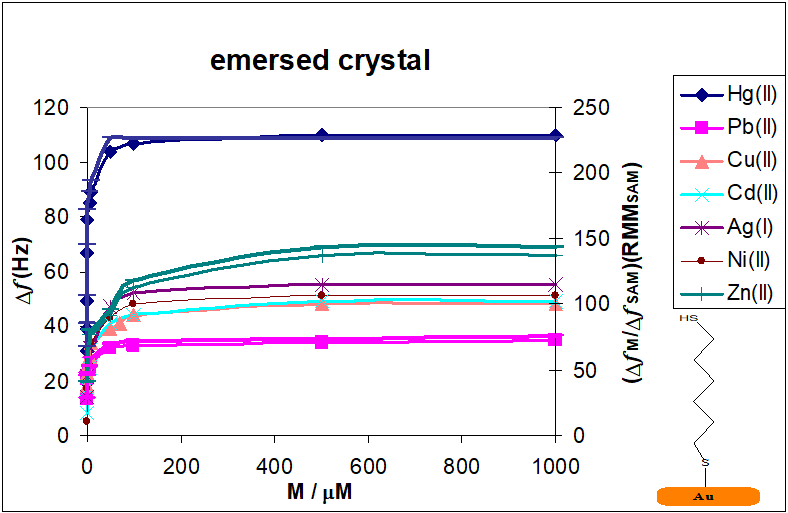
Figure 5: The binding isotherms for the binding Hg2+, Cd2+, Pb2+, Cu2+, Ni2+, Zn2+ and Ag+ to 1,6-hexanedithiol SAM on emersed crystal. The surface coverage of the monolayer was 3.14 nmol cm-2.
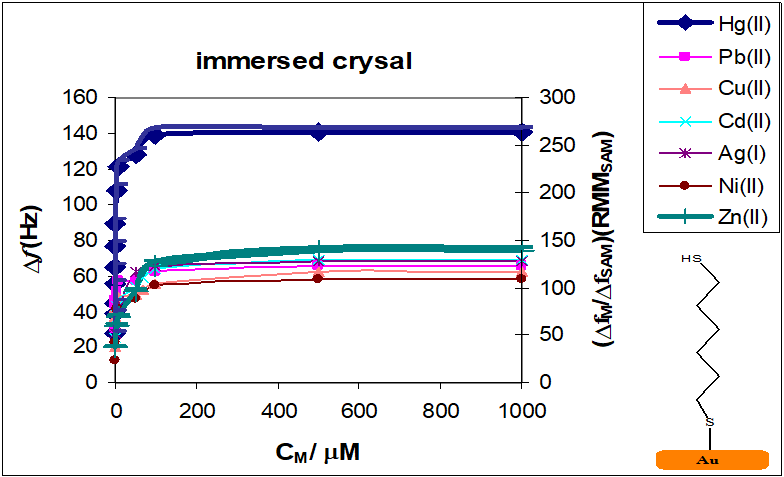
Figure 6: The binding isotherms for the binding Hg2+, Cd2+, Pb2+, Cu2+, Ni2+, Zn2+ and Ag+ to1,6-hexanedithol SAM on immersed crystal. The surface coverage of the monolayer was 3.33 nmol cm-2.
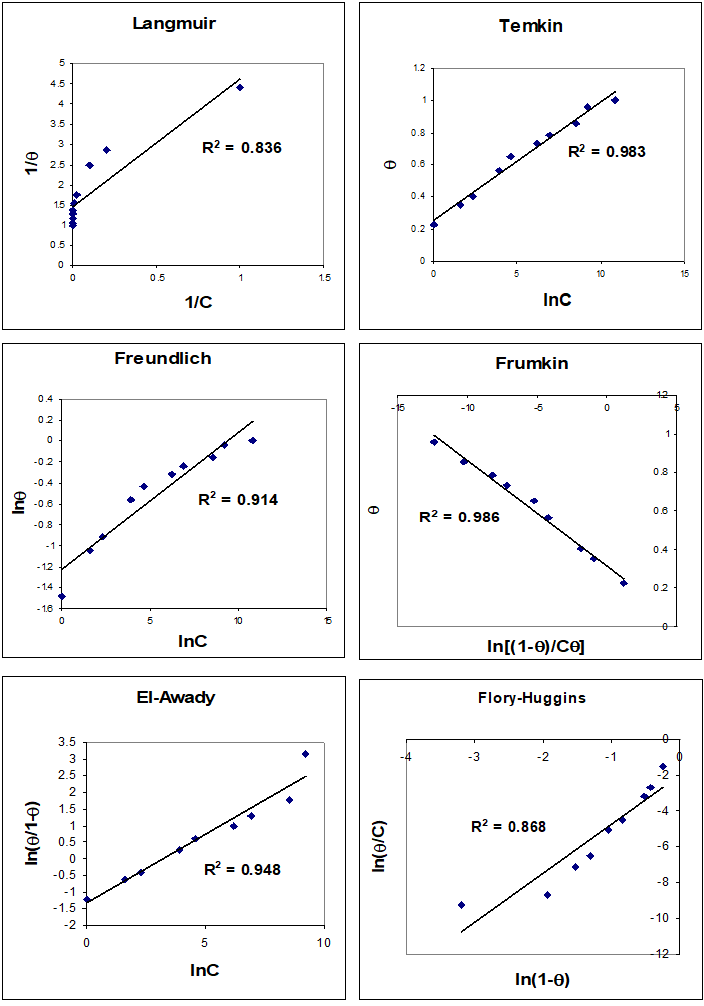
Figure 7: Plot of Langmuir, Temkin, Freundlich, Frumkin, El-Awady and Flory-Huggins isotherms to estimate the binding constant between Hg2+ and 1,6-hexanedithol on dry (emersed) crystal. The line represents the linear least squares fit to the experimental data (points)
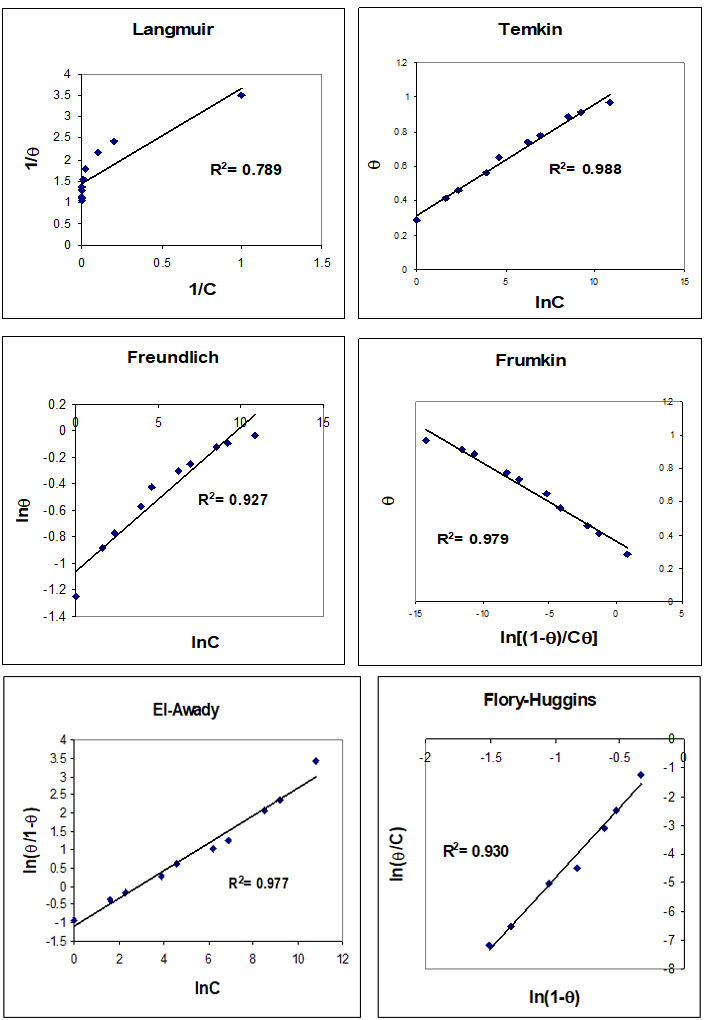
Figure 8: Plot of Langmuir, Temkin, Freundlich, Frumkin, El-Awady and Flory-Huggins isotherms to estimate the binding constant between Hg2+ and 1,6-hexanedithol on wet (immersed) crystal. The line represents the linear least squares fit to the experimental data (points).
lnK | Hg2+ | Cd2+ | Pb2+ | Zn2+ | Cu2+ | Ni2+ |
lnKT Dry n=4 | 21.1±0.04 | 15.4±1.00 | 14.02±0.09 | 16.5±0.04 | 13.0±0.2 | 14.1±0.2 |
lnKT Wet n=4 | 21.0±0.09 | 14.8±0.8 | 13.9±0.04 | 16.1±0.03 | 13.2±0.3 | 13.9±0.1 |
Table 1: Equilibrium binding constants for different metal ions with 1, 6-hexanedithiol were estimated from the linearized form of the Temkin adsorption isotherm.
One can see from the results of the binding constants of the ligand 1,6-hexedithiol with different metal ions, the ligand has selectivity to Hg(II) from aqueous solutions over the other metal ions. The order of binding constants is Hg (II)> Zn (II)> Cd (II)> Pb(II)> Ni(II)> Cu(II)> Ag(I).
Effect of the pH on the complexation between Hg (II) and HDT
The effect of the pH on the complexation between Hg (II) ions and HDT was investigated using the same procedures described above. The pH of the solutions was adjusted with 0.1 M KOH or 0.1 M HNO3. The relationship between the pH and the mole ratio between HDT and Hg (II) is shown in Figure 9. It was found that the mole ratio increased rapidly with increasing pH of the solution. At pH around five, the mole ratio between HDT and Hg (II) is around one. The mole ratio between HDT/Hg (II) is changed from one to three at higher pH values. The –SH groups are protonated at low pH values; thus, it is not free (suitable) to complex the mercury ions from solution. On the other hand, mercury hydroxides were formed at high pH values (pH≥6) and the mole ratio was changed as a result of formation new species in solution. The best observed pH was around 4.5-5.0, which gives a good compromise between Hg(II) and HDT ligand availability and all –SH groups are deprotonated in this range. It is important to note that no buffer systems have been used to control the pH of the preconcentration solution because most of buffer systems adsorbed on the Au of the quartz crystal and may interfere with the detection [27].
The effect of the pH on the complexation between Hg (II) ions and HDT was investigated using the same procedures described above. The pH of the solutions was adjusted with 0.1 M KOH or 0.1 M HNO3. The relationship between the pH and the mole ratio between HDT and Hg (II) is shown in Figure 9. It was found that the mole ratio increased rapidly with increasing pH of the solution. At pH around five, the mole ratio between HDT and Hg (II) is around one. The mole ratio between HDT/Hg (II) is changed from one to three at higher pH values. The –SH groups are protonated at low pH values; thus, it is not free (suitable) to complex the mercury ions from solution. On the other hand, mercury hydroxides were formed at high pH values (pH≥6) and the mole ratio was changed as a result of formation new species in solution. The best observed pH was around 4.5-5.0, which gives a good compromise between Hg(II) and HDT ligand availability and all –SH groups are deprotonated in this range. It is important to note that no buffer systems have been used to control the pH of the preconcentration solution because most of buffer systems adsorbed on the Au of the quartz crystal and may interfere with the detection [27].
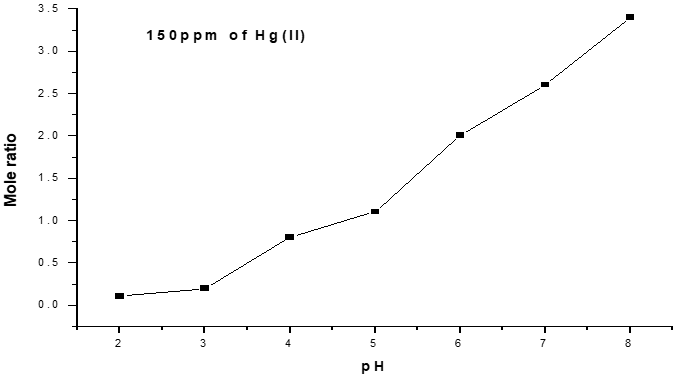
Figure 9: pH dependence on the adsorption of Hg (II) ions onto HDT SAM modified Au quartz crystal (immersed crystal). The concentration of the Hg (II) was 20.0 ppm.
1,6-hexanedithiol-Pt-QCM-Au NPs to analyse mixtures of metal ions
In the first stage, the concentration of mercury ions in binary mixtures (Hg2+,Cu2+), (Hg2+,Cd2+), (Hg2+,Zn2+2+), (Hg2+, Ni2+), (Hg2+, Pb2+) and (Hg2+, Ag2++) was fixed at 0.1 mM and the concentration of interfering ions was varied from 0.1 mM to 9 mM. In the second stage the concentration of Hg (II) was varied from 1 μM to 1 mM, with the concentrations of interfering ions fixed at 1mM. The results of the two stages are shown in Figures 10 and 11. The results show that the frequency shifts for Hg (II) in binary mixtures in both cases are essentially the same range as the frequency shifts for single mercury ion solutions. Thus, these interfering ions have no significant influence on the Hg (II) determination with 1,6-hexanedithiol SAM modified Au NPs electrode.
In the first stage, the concentration of mercury ions in binary mixtures (Hg2+,Cu2+), (Hg2+,Cd2+), (Hg2+,Zn2+2+), (Hg2+, Ni2+), (Hg2+, Pb2+) and (Hg2+, Ag2++) was fixed at 0.1 mM and the concentration of interfering ions was varied from 0.1 mM to 9 mM. In the second stage the concentration of Hg (II) was varied from 1 μM to 1 mM, with the concentrations of interfering ions fixed at 1mM. The results of the two stages are shown in Figures 10 and 11. The results show that the frequency shifts for Hg (II) in binary mixtures in both cases are essentially the same range as the frequency shifts for single mercury ion solutions. Thus, these interfering ions have no significant influence on the Hg (II) determination with 1,6-hexanedithiol SAM modified Au NPs electrode.
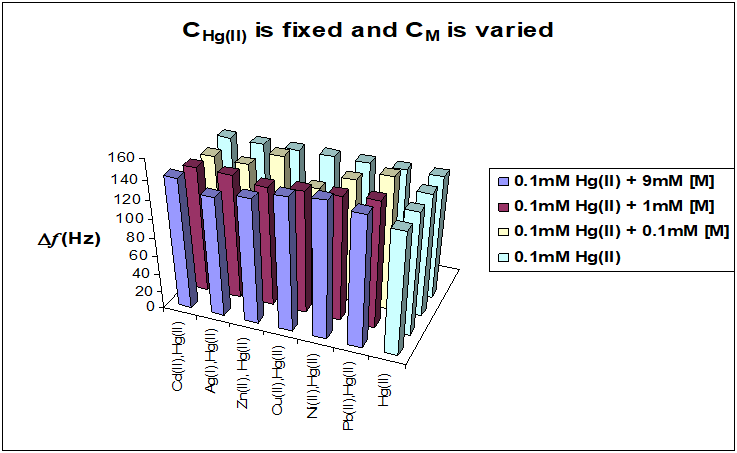
Figure 10: Comparison between the adsorption Hg (II) ions from single ion solutions and binary mixtures with interfering ions. The concentration of interfering metal ions was varied from 0.1 mM to 9 mM and the concentration of Hg (II) was fixed at 0.1 mM in all cases. The same surface coverage of the ligand was used, 1.66 nmol cm-2.
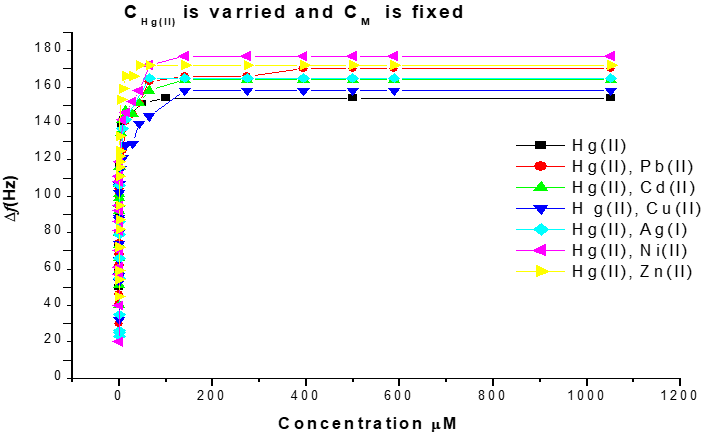
Figure 11: Comparison between experimental adsorption isotherms for mercury ions from single metal solution and binary mixtures with 1,6-hexanedithiol, where the concentrations of interfering ions were fixed to 1 mM. The surface coverage of ligand was 1.70 nmol cm-2.
X-Ray photoelectron spectroscopy (XPS) is a well-established tool for the characterization of the molecular structure of organic films. XPS survey spectra can be used to confirm the presence of desired elements in the film and to evaluate its atomic composition [28,29]. Peak positions can give information about the local environment and degree of packing of the chains in the film. Atomic ratios have to be interpreted with great care since photoelectrons from atoms near the substrate are attenuated by overlaying material. The measured atomic composition is thus sensitive to the elemental distribution perpendicular to the surface in addition to the actual composition of the film. High resolution X-ray photoelectron spectroscopy has often been used to identify the interaction of a metal ion with the surface chemical groups on an adsorbent material during adsorption because the creation of a chemical bond between a metal ion and an atom on surface of the adsorbent changes the distribution of the electrons around the corresponding atoms. The electron donating ligand can lower the binding energy (BE) of the core level electrons while electron-withdrawing ligands can increase the BE [30-32]. The XPS technique will allow us to explore both the Au/SAM and SAM/analyte interfaces, providing insight into adsorption stoichiometry and metal ion speciation.
When HDT SAM on Au NPs exposed to solutions of trace metal ions a preconcentration equilibrium is set up at electrode solution interface. The nature of the interfacial binding process will be explaining, which controls the interfacial composition, and thereby sensor performance. Using the QCM in gravimetric mode we have determined binding constants of different metal species e.g. Cu2+, Pb2+, Cd2+ and Hg2+, in single and mixed ion solutions. This is a thermodynamic probe of the interface. Similarly, we have used EQCM in coulometric mode (by integrating the voltammetric responses of surface-bound complexed metal ions) to determine metal ion coverage. However, neither of these population-based techniques provides an insight into the nature of the metal species and ligand within the complex. High resolution XPS gives us in order to acquire quantitative correlations of surface composition and structure at the interface. Thus, XPS to probes the nature of adsorbed metal ion; it’s coordination environment, oxidation state, and that of the underlying SAM as a function of electrode potential. XPS gives more information about the preconcentration equilibria (particularly under competitive situations) and metal speciation at HDT SAMs exposed to dilute aqueous solutions of single and multiple metal ions. The important questions to be answered using XPS are the mole ratio between the metal ion and HDT SAM ligand (S:M) (different free terminal groups) for all metal ions and the chemical changes occurring in the oxidation states of sulphur, carbon and oxygen before and after complexation with metal ions . Finally, ex situ high resolution X-ray photoelectron spectroscopy (XPS) calibrates the results that were extracted before from QCM-EQCM measurements.
Preliminary survey spectra scans of the HDT SAM-modified Au NPs indicated the presence of all the expected elements (viz., C, S, O, Au and also Hg (II) metal but no extraneous elements were observed. These results confirmed that the Au NPs surfaces used for the monolayer preparations and for monolayers with Hg (II) were of high purity and did not have any significant amount of contamination. The results of the survey spectra and high-resolution spectra for the elements in the SAMs of 1,6-hexanedithiol HDT before and after adsorbed Hg2+, respectively, are shown in Figures 12 and 13. The XPS spectra in these figures showed characteristic peaks corresponding to C (1s) (285 eV), O (1s) (532 eV), S (2p3/2) (162 eV) and Hg (4f7/2).).
The atom percent of carbon, oxygen, sulphur, and Hg (II) metal ions were calculated from the high resolution spectra collected at normal emission by integration of the areas of the S (2p3/2), C1s, O1s, and Hg (4f7/2) peaks after application of a linear background subtraction [33] over a suitable range encompassing the peaks, and correction using the atomic sensitivity factors. The atomic sensitivity factors employed in this study for comparison of relative atomic concentration in HDT on Au NPs were provided by the instrumental manufacturer and are as follows: S(2p3/2) = 2.08, C1s =1.00, O1s = 2.80, and Hg(4f7/2) = 10.57. The results of the elemental analysis are summarized in tables 2.
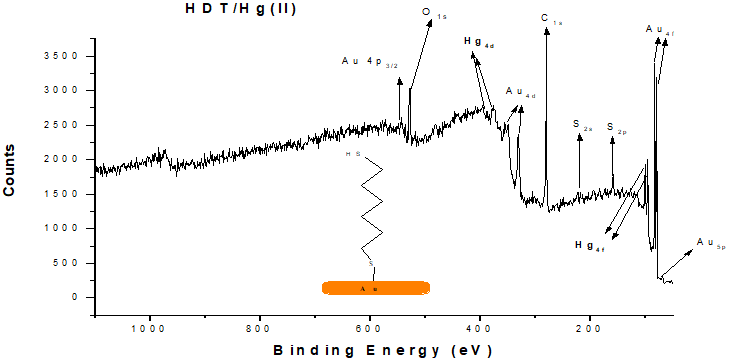
Figure 12: XPS wide scan spectra of a SAM of 1,6-hexanedithiol HDT after a preconcentration step in 1mM mercury nitrate solution (pH 5.0) for 20 min followed by high resolution XPS scans for C1s, O1s, Au(4f7/2), S(2p3/2), and Hg(4f7/2).
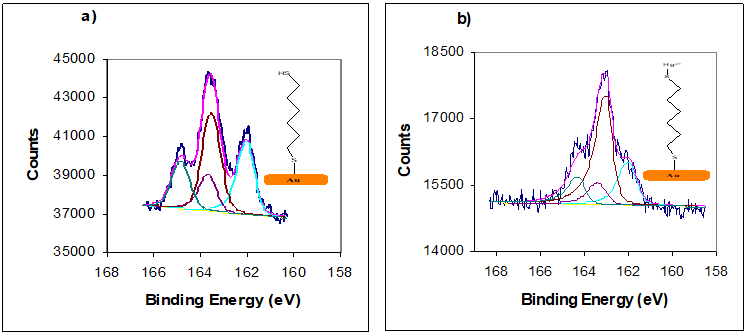
Figure 13: XPS spectra in S (2p) SAMs of (a) HDT; (b) HDT/Hg (II);). All the SAMs were prepared by immersion for 24h into 1mM solution. Two S2p doublets, both with a 2:1 area ratio and a splitting of 1.5 eV, were used to peak fit the experimental spectrum.
SAMs / M | Name | Area (cps) | Sensitivity | Atom. (%) | Hg:S |
HDT | C 1s | 3679 | 1.00 | 77.79 | |
HDT | O 1s | 1862 | 2.80 | 14.06 | |
HDT | S 2p3/2 | 534 | 2.08 | 5.42 | |
HDT/Hg(II) | Hg 4f7/2 | 1364 | 10.57 | 4.73 | 0.88 |
Table 2: Elemental composition of SAM of hexandithiol HDT on Au NPs (obtained from high resolution XPS spectra) after adsorbing Hg (II) ions from single metal ion solutions and the concentration of the three metal ions was 1mM. Table also shows elemental composition of SAM of 1,6-hexanedithiol HDT after adsorbing Hg2+ from single ion solution.
The relative concentration of the detected Hg (II) ions was used to determine the metal ion to sulphur ratio are presented in table 2. The mole ratios were estimated after exposure to high concentrations of Hg (II) ion, as in the QCM and EQCM experiments. The mole ratios extracted from the XPS analyse are in agreement with the mole ratios extracted from QCM and EQCM measurements. The binding energy 163.6-164 eV for free -SH was observed only for 1,6-hexanedithiol HDT (see Figures 12 and 13) before adsorption of Hg(II) ions and disappeared after adsorption of Hg(II) from aqueous solutions. Based on the peak area ratios, ratio of the two chemical states of sulfur, XPS data suggest that one-ended dithiol molecules (HDT) attached to the Au substrate to form AuSR and the other ended thiol groups were left as free -SH, which is consistent with previous studies for 1-5-pentanedithiol and 1,9-nonanedithiol on Au electrodes [34,35] (see table 2). The curve-fitted high resolution XPS spectra obtained for the C(1s) binding energy region present three peaks in the 284.3, 284.9 and 286.3 eV .The peaks are assigned from literature references to the –C-C, CH2-CH2, and-C-S, respectively [36,37,38].
Conclusions
Mercury ion selective acoustic wave nano sensor method was developed and uses the Pt-QCM technique after modification with Au NPs with 1,6-hexanedithiol SAMs. The binding of different concentrations of Hg2+ and interfering metal ions to the same ligand was also studied, including the estimation of binding stoichiometry between the SAMs and Hg2+. The combination of SAM selectivity and QCM sensitivity provides a potentially useful trace metal ion acoustic wave nano sensor in the micro molar range.
1,6-hexanedithio SAMs modified Au NPs promoted the adsorption of mercury ions at pH=5.0-5.5. The response to Hg+2 at concentrations 10μM-10mM was fitted to Langmuir, Temkin, Frumkin, Freundlich, El-Awady and Flory-Huggins isotherms. The data fit very well to the Temkin and Frumkin isotherms. From the slope and intercept of the linear regression of these isotherms on dry (emersed) and wet (immersed) crystals, it was possible to estimate the binding constant between the Hg2+ and the ligand. To study the selectivity and sensitivity of the ligand, other metal ions such as Pb2+, Cd2+, Cu2+, Zn+2, Ni2+ and Ag+ were bound to the HDT monolayer and the same methodology was used for binding other metal ions. It was found that the ligand has a strong selectivity to Hg2+ and the adsorption of these metal ions to the ligand followed the sequence Hg(II)> Zn(II)> Cd(II)> Pb(II)> Ni(II)> Cu(II)> Ag(I). The stoichiometry between the Hg2+ and the ligand is (1:1). SAM of 1,6-hexanedithiol modified Au electrodes has a high selectivity to Hg(II) ions.
The uptake of interfering metal ions and Hg2+ from binary mixtures using same ligand has been developed using two procedures. In the first procedure the concentration of Hg (II) metal ions was fixed and the concentration of the interfering metal ion in the binary mixture was varied. Comparison was made between the frequency shift for the target metal ions in the single ion solution and in the binary mixture. It was found that the frequency shift for the target metal ion in single metal ions was essentially the same as in the binary mixtures. One can conclude that the second metal ion has no significant influence on the determination of target metal ion. In the second procedure the concentration of target metal ion in binary mixture was varied with the interfering metal ion concentration fixed. The adsorption isotherm of the target metal ion was fitted to six kinds of isotherms and it was found that the Frumkin and Temkin isotherms fitted the experimental data best. When comparing the values of binding constant for target metal ions in single solution to the binary mixtures. The results presented for high resolution XPS elemental composition for HDT and HDT/Hg2+ show the presence of sulphur, carbon, oxygen, gold and target metal ions. These results also confirmed that HDT are successfully immobilized on Au NPs and can bind different metal ions from aqueous solutions with high selectivity to Hg (II) ions.
Acknowledgments
The authors acknowledge the finical support of this work from Authority of Natural Science Research and Technology (ANSRT).
The authors acknowledge the finical support of this work from Authority of Natural Science Research and Technology (ANSRT).
References
- L.Sartore, M.Barbaylio.L.Borgese and E.Bontempi, Sensors and Actuators B, 155, (2011), 538.
- A.M.Etorki,A.R.Hillman, K.S.Ryder and A.Glidle, J.Electroanal.Chem, 599, (2007),275.
- L.Eddaif, A.shaban and J.Telegdi,Int.J.Environ.Anal.Chem, 99,9 ,(2019), 824.
- Widmann.A, Van der Berg.C.G, Electroanalysis, 17, 10, (2005), 825-831.
- Turyan.I, Mandler. D, Analytical Chemistry, 69, (1997), 894-897.
- Berchmans.S, Arivukkodi.S, Yegnaraman.V, Electrochemistry Communications, 2, (2000), 226.
- Herzog.G, Arrigan.D.W.M, Analytical Chemistry, 75, 2, (2003), 319-323.
- Wang.S.F, Du.D, Zoc.Q. C., Acta Physico-Chimica Sinica, 17, (2001), 1102-1106.
- Turyan.I, Mandler.D, Analytical chemistry, 66, 1, (1994), 58-63.
- Herzog.G, Arrigan.G.D.W, Electroanalysis, 15-16, (2003), 1302-1306.
- H.Boom,H.Li and S.F.Yau.Li,Analyst,139,(2014), 5170.
- Z.Mu.Dong and J.C.Zhao, Sensors, 12, (2012), 7080.
- H.Lou, Y.Zhang, Q.Xiang, J.Xu, H.Li, P.Xu and X.Li,Sensors and Actuators B, 166-167, (2012),246.
- S.Chah, J.Yi and R.N.Zara, Sensors and Actuators B, 99 (2004), 216.
- W.M.E.M.Daniyal, S.Saleviter and Y.W.Fen, Sensors and Materials, 30, 9, (2018), 2023.
- T.Kang, J.Moon, S.Oh, S.Hong, S.Chah and J.Yi, Chem.Commun, (2005), 2360.
- A.Bibby and L.Mercier, Chem. Mater.14, (2002), 1591.
- J.Brown, L.Mercier and T.J.Pinnavaia, Chem. Commun. (1999), 69.
- W.Deng, L.Yang, D.Fujita, H.Nejoh and C.Bai, Appl. Phys. A., 71, (2000), 639.
- M.J.Esplandiu, Probe Microscopy. 2, (2001), 89.
- T.T.Calam and E.Hasdemir, GU.J.Sci, 31, 1 (2018), 53.
- HF.Ji,Y.Zhang,VV.Purushotham, S.Kondu,B.Ramachandran,T.Thundat, and DT.Hagnie,Analyst, 130,(2005),1577.
- Y. Jin, Y.Huang, G.Liu and R.Zhao,Analyst 138,(2013),5479.
- A.M.Etorki, F.M.N.Massoudi and M.M.Abuein,Advanced Science Letters,17,1, (2012),87.
- N.Elgrishi, KJ. Rountree, BO. McCarthy, ES.Rountree, T.T. Eisenhart and JL.Dempsey, J.Chem.Educ, 95, 2, (2018), 197.
- D.Johannsmann, the Quartz Crystal Microbalance in Soft Matter Research, Fundamentals and Modeling (2015), Springer.
- C.Mizuno, S.Bao, T.Hinoue and T.Nomura, Analytical Sciences, 21, (2005), 281.
- H.L.Zhang, S.D.Evans and K.Critchley, J. Chem. Physics., 122, (2005), 224707.
- K.D.Weldige, M.Rohwerder, E.Vago, H.Viefhaus and M.Stratmann, Fresenius J Anal Chem., 353, (1995), 329
- X.Zhang and R.B.Bai, J.Mater. Chem, 12, (2002), 2733.
- M.S.Polo and J.R.Utrilla, Environ.Sci. Technol., 36, (2002), 3850.
- A.S.Duwez, J.Electr.Spectrosc.Relat. Phenom, 134, (2004), 97.
- M.Zharnikov. J.Electr.Spectrosc.Relat. Phenom.178-179,(2010), 380.
- W.Deng, D.Fujita, L.Yang, H.Nejo and C.Bai, Jpn.J.Appl.Phys. 39, (2000), L751.
- W.Deng, L.Yang, D.Fujita, H.Nejoh and C.Bai, Appl.Phys.A. 71, (2000), 639.
- D.M.Wieliczka, J.Vac.Sci.Technol.A, 24, 5, (2006), 1756.
- R.Brito, R.Tremont, O.Feliciano and C.R.Cabrera, J.Electroanal.Chem. 540, (2003), 53.
- G.Greczynsk and L.Hultman, Progress in Material Science, 107, (2020), 10059.
Citation: AM Etorki, M. El-Rais and MS Elhabbat. (2020). Development of an Acoustic wave Nano Sensor for Hg(II) using Quartz Crystal Microbalance Modified with gold Nanoparticles- 1,6-hexanedithiol. Archives of Chemistry and Chemical Engineering 2(1).
Copyright: © 2020 Abdunnaser Etorki. This is an open-access article distributed under the terms of the Creative Commons Attribution License, which permits unrestricted use, distribution, and reproduction in any medium, provided the original author and source are credited.